19 The Nervous System
Original chapter by Aneeq Ahmad adapted by the Queen’s University Psychology Department
This Open Access chapter was originally written for the NOBA project. Information on the NOBA project can be found below.
We encourage students to use the “Three-Step Method” for support in their learning. Please find our version of the Three-Step Method, created in collaboration with Queen’s Student Academic Success Services, at the following link: https://sass.queensu.ca/psyc100/
The mammalian nervous system is a complex biological organ, which enables many animals including humans to function in a coordinated fashion. The original design of this system is preserved across many animals through evolution; thus, adaptive physiological and behavioral functions are similar across many animal species. Comparative study of physiological functioning in the nervous systems of different animals lend insights to their behavior and their mental processing and make it easier for us to understand the human brain and behavior. In addition, studying the development of the nervous system in a growing human provides a wealth of information about the change in its form and behaviors that result from this change. The nervous system is divided into central and peripheral nervous systems, and the two heavily interact with one another. The peripheral nervous system controls volitional (somatic nervous system) and nonvolitional (autonomic nervous system) behaviors using cranial and spinal nerves. The central nervous system is divided into forebrain, midbrain, and hindbrain, and each division performs a variety of tasks; for example, the cerebral cortex in the forebrain houses sensory, motor, and associative areas that gather sensory information, process information for perception and memory, and produce responses based on incoming and inherent information. To study the nervous system, a number of methods have evolved over time; these methods include examining brain lesions, microscopy, electrophysiology, electroencephalography, and many scanning technologies.
Learning Objectives
- Describe and understand the development of the nervous system.
- Learn and understand the two important parts of the nervous system.
- Explain the two systems in the peripheral nervous system and what you know about the different regions and areas of the central nervous system.
- Learn and describe different techniques of studying the nervous system. Understand which of these techniques are important for cognitive neuroscientists.
- Describe the reasons for studying different nervous systems in animals other than human beings. Explain what lessons we learn from the evolutionary history of this organ.
Evolution of the Nervous System
Many scientists and thinkers (Cajal, 1937; Crick & Koch, 1990; Edelman, 2004) believe that the human nervous system is the most complex machine known to man. Its complexity points to one undeniable fact—that it has evolved slowly over time from simpler forms. Evolution of the nervous system is intriguing not because we can marvel at this complicated biological structure, but it is fascinating because it inherits a lineage of a long history of many less complex nervous systems (Figure 1), and it documents a record of adaptive behaviors observed in life forms other than humans. Thus, evolutionary study of the nervous system is important, and it is the first step in understanding its design, its workings, and its functional interface with the environment.

The brains of some animals, like apes, monkeys, and rodents, are structurally similar to humans (Figure 1), while others are not (e.g., invertebrates, single-celled organisms). Does anatomical similarity of these brains suggest that behaviors that emerge in these species are also similar? Indeed, many animals display behaviors that are similar to humans, e.g., apes use nonverbal communication signals with their hands and arms that resemble nonverbal forms of communication in humans (Gardner & Gardner, 1969; Goodall, 1986; Knapp & Hall, 2009). If we study very simple behaviors, like physiological responses made by individual neurons, then brain-based behaviors of invertebrates (Kandel & Schwartz, 1982) look very similar to humans, suggesting that from time immemorial such basic behaviors have been conserved in the brains of many simple animal forms and in fact are the foundation of more complex behaviors in animals that evolved later (Bullock, 1984).
Even at the micro-anatomical level, we note that individual neurons differ in complexity across animal species. Human neurons exhibit more intricate complexity than other animals; for example, neuronal processes (dendrites) in humans have many more branch points, branches, and spines.
Complexity in the structure of the nervous system, both at the macro- and micro-levels, give rise to complex behaviors. We can observe similar movements of the limbs, as in nonverbal communication, in apes and humans, but the variety and intricacy of nonverbal behaviors using hands in humans surpasses apes. Deaf individuals who use American Sign Language (ASL) express themselves in English nonverbally; they use this language with such fine gradation that many accents of ASL exist (Walker, 1987). Complexity of behavior with increasing complexity of the nervous system, especially the cerebral cortex, can be observed in the genus Homo (Figure 2). If we compare sophistication of material culture in Homo habilis (2 million years ago; brain volume ~650 cm3) and Homo sapiens (300,000 years to now; brain volume ~1400 cm3), the evidence shows that Homo habilis used crude stone tools compared with modern tools used by Homo sapiens to erect cities, develop written languages, embark on space travel, and study her own self. All of this is due to increasing complexity of the nervous system.
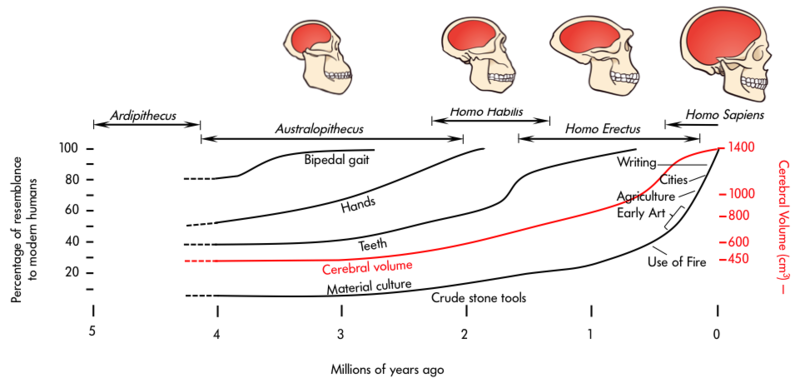
What has led to the complexity of the brain and nervous system through evolution, to its behavioral and cognitive refinement? Darwin (1859, 1871) proposed two forces of natural and sexual selection as work engines behind this change. He prophesied, “psychology will be based on a new foundation, that of the necessary acquirement of each mental power and capacity by gradation” that is, psychology will be based on evolution (Rosenzweig, Breedlove, & Leiman, 2002).
Development of the Nervous System
Where the study of change in the nervous system over eons is immensely captivating, studying the change in a single brain during individual development is no less engaging. In many ways the ontogeny (development) of the nervous system in an individual mimics the evolutionary advancement of this structure observed across many animal species. During development, the nervous tissue emerges from the ectoderm (one of the three layers of the mammalian embryo) through the process of neural induction. This process causes the formation of the neural tube, which extends in a rostrocaudal (head-to-tail) plane. The tube, which is hollow, seams itself in the rostrocaudal direction. In some disease conditions, the neural tube does not close caudally and results in an abnormality called spina bifida. In this pathological condition, the lumbar and sacral segments of the spinal cord are disrupted.
As gestation progresses, the neural tube balloons up (cephalization) at the rostral end, and forebrain, midbrain, hindbrain, and the spinal cord can be visually delineated (day 40). About 50 days into gestation, six cephalic areas can be anatomically discerned (also see below for a more detailed description of these areas).
The progenitor cells (neuroblasts) that form the lining (neuroepithelium) of the neural tube generate all the neurons and glial cells of the central nervous system. During early stages of this development, neuroblasts rapidly divide and specialize into many varieties of neurons and glial cells, but this proliferation of cells is not uniform along the neural tube—that is why we see the forebrain and hindbrain expand into larger cephalic tissues than the midbrain. The neuroepithelium also generates a group of specialized cells that migrate outside the neural tube to form the neural crest. This structure gives rise to sensory and autonomic neurons in the peripheral nervous system.
The Structure of the Nervous System
The mammalian nervous system is divided into central and peripheral nervous systems.
The Peripheral Nervous System
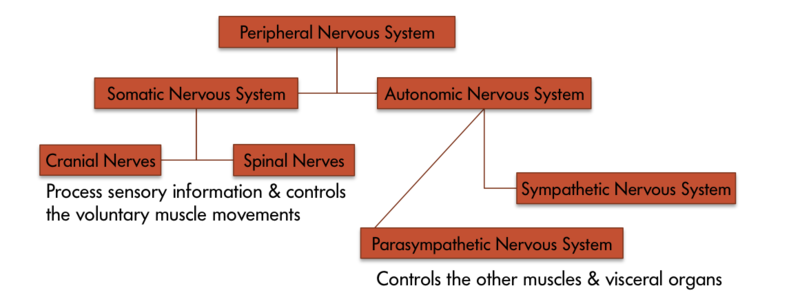
The peripheral nervous system is divided into somatic and autonomic nervous systems (Figure 3). Where the somatic nervous system consists of cranial nerves (12 pairs) and spinal nerves (31 pairs) and is under the volitional control of the individual in maneuvering bodily muscles, the autonomic nervous system also running through these nerves lets the individual have little control over muscles and glands. Main divisions of the autonomic nervous system that control visceral structures are the sympathetic and parasympathetic nervous systems.
At an appropriate cue (say a fear-inducing object like a snake), the sympathetic division generally energizes many muscles (e.g., heart) and glands (e.g., adrenals), causing activity and release of hormones that lead the individual to negotiate the fear-causing snake with fight-or-flight responses. Whether the individual decides to fight the snake or run away from it, either action requires energy; in short, the sympathetic nervous system says “go, go, go.” The parasympathetic nervous system, on the other hand, curtails undue energy mobilization into muscles and glands and modulates the response by saying “stop, stop, stop.” This push–pull tandem system regulates fight-or-flight responses in all of us.
The Central Nervous System
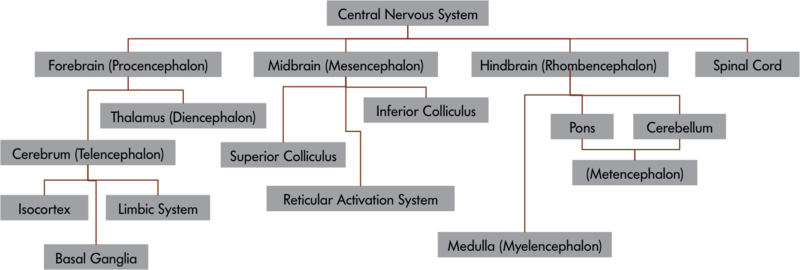
The central nervous system is divided into a number of important parts (see Figure 4), including the spinal cord, each specialized to perform a set of specific functions. Telencephalon or cerebrum is a newer development in the evolution of the mammalian nervous system. In humans, it is about the size of a large napkin and when crumpled into the skull, it forms furrows called sulci (singular form, sulcus). The bulges between sulci are called gyri (singular form, gyrus). The cortex is divided into two hemispheres, and each hemisphere is further divided into four lobes (Figure 5a), which have specific functions. The division of these lobes is based on two delineating sulci: the central sulcus divides the hemisphere into frontal and parietal-occipital lobes and the lateral sulcus marks the temporal lobe, which lies below.
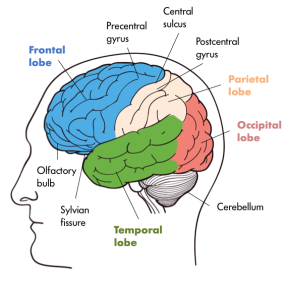
Just in front of the central sulcus lies an area called the primary motor cortex (precentral gyrus), which connects to the muscles of the body, and on volitional command moves them. From mastication to movements in the genitalia, the body map is represented on this strip (Figure 5b).
Some body parts, like fingers, thumbs, and lips, occupy a greater representation on the strip than, say, the trunk. This disproportionate representation of the body on the primary motor cortex is called the magnification factor (Rolls & Cowey, 1970) and is seen in other motor and sensory areas. At the lower end of the central sulcus, close to the lateral sulcus, lies the Broca’s area (Figure 6b) in the left frontal lobe, which is involved with language production. Damage to this part of the brain led Pierre Paul Broca, a French neuroscientist in 1861, to document many different forms of aphasias, in which his patients would lose the ability to speak or would retain partial speech impoverished in syntax and grammar (AAAS, 1880). It is no wonder that others have found subvocal rehearsal and central executive processes of working memory in this frontal lobe (Smith & Jonides, 1997, 1999).
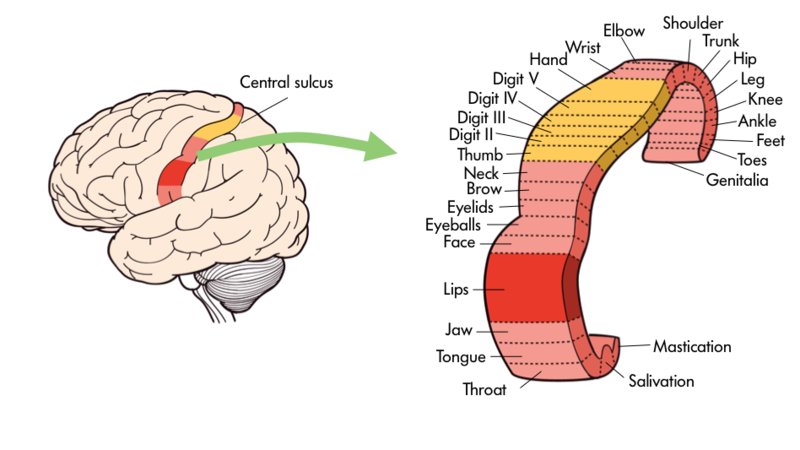
Just behind the central gyrus, in the parietal lobe, lies the primary somatosensory cortex (Figure 6a) on the postcentral gyrus, which represents the whole body receiving inputs from the skin and muscles. The primary somatosensory cortex parallels, abuts, and connects heavily to the primary motor cortex and resembles it in terms of areas devoted to bodily representation. All spinal and some cranial nerves (e.g., the facial nerve) send sensory signals from skin (e.g., touch) and muscles to the primary somatosensory cortex. Close to the lower (ventral) end of this strip, curved inside the parietal lobe, is the taste area (secondary somatosensory cortex), which is involved with taste experiences that originate from the tongue, pharynx, epiglottis, and so forth.
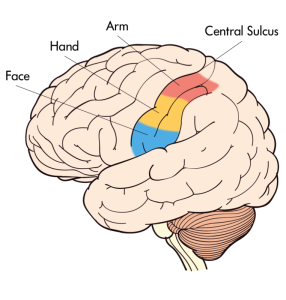
Just below the parietal lobe, and under the caudal end of the lateral fissure, in the temporal lobe, lies the Wernicke’s area (Demonet et al., 1992). This area is involved with language comprehension and is connected to the Broca’s area through the arcuate fasciculus, nerve fibers that connect these two regions. Damage to the Wernicke’s area (Figure 6b) results in many kinds of agnosias; agnosia is defined as an inability to know or understand language and speech-related behaviors. So an individual may show word deafness, which is an inability to recognize spoken language, or word blindness, which is an inability to recognize written or printed language. Close in proximity to the Wernicke’s area is the primary auditory cortex, which is involved with audition, and finally the brain region devoted to smell (olfaction) is tucked away inside the primary olfactory cortex (prepyriform cortex).
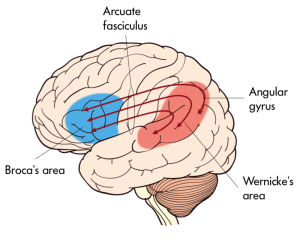
At the very back of the cerebral cortex lies the occipital lobe housing the primary visual cortex. Optic nerves travel all the way to the thalamus (lateral geniculate nucleus, LGN) and then to visual cortex, where images that are received on the retina are projected (Hubel, 1995).In the past 50 to 60 years, visual sense and visual pathways have been studied extensively, and our understanding about them has increased manifold. We now understand that all objects that form images on the retina are transformed (transduction) in neural language handed down to the visual cortex for further processing. In the visual cortex, all attributes (features) of the image, such as the color, texture, and orientation, are decomposed and processed by different visual cortical modules (Van Essen, Anderson & Felleman, 1992) and then recombined to give rise to singular perception of the image in question.If we cut the cerebral hemispheres in the middle, a new set of structures come into view. Many of these perform different functions vital to our being. For example, the limbic system contains a number of nuclei that process memory (hippocampus and fornix) and attention and emotions (cingulate gyrus); the globus pallidus is involved with motor movements and their coordination; the hypothalamus and thalamus are involved with drives, motivations, and trafficking of sensory and motor throughputs. The hypothalamus plays a key role in regulating endocrine hormones in conjunction with the pituitary gland that extends from the hypothalamus through a stalk (infundibulum).
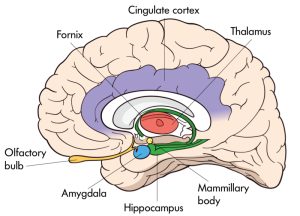
As we descend down the thalamus, the midbrain comes into view with superior and inferior colliculi, which process visual and auditory information, as does the substantia nigra, which is involved with notorious Parkinson’s disease, and the reticular formation regulating arousal, sleep, and temperature. A little lower, the hindbrain with the pons processes sensory and motor information employing the cranial nerves, works as a bridge that connects the cerebral cortex with the medulla, and reciprocally transfers information back and forth between the brain and the spinal cord. The medulla oblongata processes breathing, digestion, heart and blood vessel function, swallowing, and sneezing. The cerebellum controls motor movement coordination, balance, equilibrium, and muscle tone.
The midbrain and the hindbrain, which make up the brain stem, culminate in the spinal cord. Whereas inside the cerebral cortex, the gray matter (neuronal cell bodies) lies outside and white matter (myelinated axons) inside; in the spinal cord this arrangement reverses, as the gray matter resides inside and the white matter outside. Paired nerves (ganglia) exit the spinal cord, some closer in direction towards the back (dorsal) and others towards the front (ventral). The dorsal nerves (afferent) receive sensory information from skin and muscles, and ventral nerves (efferent) send signals to muscles and organs to respond.
Studying the Nervous System
The study of the nervous system involves anatomical and physiological techniques that have improved over the years in efficiency and caliber. Clearly, gross morphology of the nervous system requires an eye-level view of the brain and the spinal cord. However, to resolve minute components, optical and electron microscopic techniques are needed.
Light microscopes and, later, electron microscopes have changed our understanding of the intricate connections that exist among nerve cells. For example, modern staining procedures (immunocytochemistry) make it possible to see selected neurons that are of one type or another or are affected by growth. With better resolution of the electron microscopes, fine structures like the synaptic cleft between the pre- and post-synaptic neurons can be studied in detail.
Along with the neuroanatomical techniques, a number of other methodologies aid neuroscientists in studying the function and physiology of the nervous system. Early on, lesion studies in animals (and study of neurological damage in humans) provided information about the function of the nervous system, by ablating (removing) parts of the nervous system or using neurotoxins to destroy them and documenting the effects on behavior or mental processes. Later, more sophisticated microelectrode techniques were introduced, which led to recording from single neurons in the animal brains and investigating their physiological functions. Such studies led to formulating theories about how sensory and motor information are processed in the brain. To study many neurons (millions of them at a time) electroencephalographic (EEG) techniques were introduced. These methods are used to study how large ensembles of neurons, representing different parts of the nervous system, with (event-related potentials) or without stimulation function together. In addition, many scanning techniques that visualize the brain in conjunction with methods mentioned above are used to understand the details of the structure and function of the brain. These include computerized axial tomography (CAT), which uses X-rays to capture many pictures of the brain and sandwiches them into 3-D models to study it. The resolution of this method is inferior to magnetic resonance imaging (MRI), which is yet another way to capture brain images using large magnets that bobble (precession) hydrogen nuclei in the brain. Although the resolution of MRI scans is much better than CAT scans, they do not provide any functional information about the brain. Positron Emission Tomography (PET) involves the acquisition of physiologic (functional) images of the brain based on the detection of positrons. Radio-labeled isotopes of certain chemicals, such as an analog of glucose (fluorodeoxyglucose), enters the active nerve cells and emits positrons, which are captured and mapped into scans. Such scans show how the brain and its many modules become active (or not) when energized with entering glucose analog. Disadvantages of PET scans include being invasive and rendering poor spatial resolution. The latter is why modern PET machines are coupled with CAT scanners to gain better resolution of the functioning brain. Finally, to avoid the invasiveness of PET, functional MRI (fMRI) techniques were developed. Brain images based on fMRI technique visualize brain function by changes in the flow of fluids (blood) in brain areas that occur over time. These scans provide a wealth of functional information about the brain as the individual may engage in a task, which is why the last two methods of brain scanning are very popular among cognitive neuroscientists.
Understanding the nervous system has been a long journey of inquiry, spanning several hundreds of years of meticulous studies carried out by some of the most creative and versatile investigators in the fields of philosophy, evolution, biology, physiology, anatomy, neurology, neuroscience, cognitive sciences, and psychology. Despite our profound understanding of this organ, its mysteries continue to surprise us, and its intricacies make us marvel at this complex structure unmatched in the universe.
A Video Exploration of the Limbic System
To help you visualize the limbic system, we recommend this video:
Studying Neurobiology in Queen’s Psychology
We have lots of opportunities for you to learn about neurons and the nervous system (including the brain, of course!) in Psychology at Queen’s. Some courses you might consider include PSYC271: Brain and Behaviour I, and PSYC370: Brain & Behaviour II.
Check Your Knowledge
To help you with your studying, we’ve included some practice questions for this module. These questions do not necessarily address all content in this module. They are intended as practice, and you are responsible for all of the content in this module even if there is no associated practice question. To promote deeper engagement with the material, we encourage you to create some questions of your own for your practice. You can then also return to these self-generated questions later in the course to test yourself.
[h5p id=”77″][h5p id=”78″][h5p id=”79″][h5p id=”80″][h5p id=”81″]
Vocabulary
- Afferent nerves
- Nerves that carry messages to the brain or spinal cord.
- Agnosias
- Due to damage of Wernicke’s area. An inability to recognize objects, words, or faces.
- Aphasia
- Due to damage of the Broca’s area. An inability to produce or understand words.
- Arcuate fasciculus
- A fiber tract that connects Wernicke’s and Broca’s speech areas.
- Autonomic nervous system
- A part of the peripheral nervous system that connects to glands and smooth muscles. Consists of sympathetic and parasympathetic divisions.
- Broca’s area
- An area in the frontal lobe of the left hemisphere. Implicated in language production.
- Central sulcus
- The major fissure that divides the frontal and the parietal lobes.
- Cerebellum
- A nervous system structure behind and below the cerebrum. Controls motor movement coordination, balance, equilibrium, and muscle tone.
- Cerebrum
- Consists of left and right hemispheres that sit at the top of the nervous system and engages in a variety of higher-order functions.
- Cingulate gyrus
- A medial cortical portion of the nervous tissue that is a part of the limbic system.
- Computerized axial tomography
- A noninvasive brain-scanning procedure that uses X-ray absorption around the head.
- Ectoderm
- The outermost layer of a developing fetus.
- Efferent nerves
- Nerves that carry messages from the brain to glands and organs in the periphery.
- Electroencephalography
- A technique that is used to measure gross electrical activity of the brain by placing electrodes on the scalp.
- A physiological measure of large electrical change in the brain produced by sensory stimulation or motor responses.
- Forebrain
- A part of the nervous system that contains the cerebral hemispheres, thalamus, and hypothalamus.
- Fornix
- (plural form, fornices) A nerve fiber tract that connects the hippocampus to mammillary bodies.
- Frontal lobe
- The most forward region (close to forehead) of the cerebral hemispheres.
- Functional magnetic resonance imaging
- (or fMRI) A noninvasive brain-imaging technique that registers changes in blood flow in the brain during a given task (also see magnetic resonance imaging).
- Globus pallidus
- A nucleus of the basal ganglia.
- Gray matter
- Composes the bark or the cortex of the cerebrum and consists of the cell bodies of the neurons (see also white matter).
- Gyrus
- (plural form, gyri) A bulge that is raised between or among fissures of the convoluted brain.
- Hippocampus
- (plural form, hippocampi) A nucleus inside (medial) the temporal lobe implicated in learning and memory.
- Homo habilis
- A human ancestor, handy man, that lived two million years ago.
- Homo sapiens
- Modern man, the only surviving form of the genus Homo.
- Hypothalamus
- Part of the diencephalon. Regulates biological drives with pituitary gland.
- Immunocytochemistry
- A method of staining tissue including the brain, using antibodies.
- Lateral geniculate nucleus
- (or LGN) A nucleus in the thalamus that is innervated by the optic nerves and sends signals to the visual cortex in the occipital lobe.
- Lateral sulcus
- The major fissure that delineates the temporal lobe below the frontal and the parietal lobes.
- Lesion studies
- A surgical method in which a part of the animal brain is removed to study its effects on behavior or function.
- Limbic system
- A loosely defined network of nuclei in the brain involved with learning and emotion.
- Magnetic resonance imaging
- Or MRI is a brain imaging noninvasive technique that uses magnetic energy to generate brain images (also see fMRI).
- Magnification factor
- Cortical space projected by an area of sensory input (e.g., mm of cortex per degree of visual field).
- Medulla oblongata
- An area just above the spinal cord that processes breathing, digestion, heart and blood vessel function, swallowing, and sneezing.
- Neural crest
- A set of primordial neurons that migrate outside the neural tube and give rise to sensory and autonomic neurons in the peripheral nervous system.
- Neural induction
- A process that causes the formation of the neural tube.
- Neuroblasts
- Brain progenitor cells that asymmetrically divide into other neuroblasts or nerve cells.
- Neuroepithelium
- The lining of the neural tube.
- Occipital lobe
- The back part of the cerebrum, which houses the visual areas.
- Parasympathetic nervous system
- A division of the autonomic nervous system that is slower than its counterpart—that is, the sympathetic nervous system—and works in opposition to it. Generally engaged in “rest and digest” functions.
- Parietal lobe
- An area of the cerebrum just behind the central sulcus that is engaged with somatosensory and gustatory sensation.
- Pons
- A bridge that connects the cerebral cortex with the medulla, and reciprocally transfers information back and forth between the brain and the spinal cord.
- Positron Emission Tomography
- (or PET) An invasive procedure that captures brain images with positron emissions from the brain after the individual has been injected with radio-labeled isotopes.
- Primary Motor Cortex
- A strip of cortex just in front of the central sulcus that is involved with motor control.
- Primary Somatosensory Cortex
- A strip of cerebral tissue just behind the central sulcus engaged in sensory reception of bodily sensations.
- Rostrocaudal
- A front-back plane used to identify anatomical structures in the body and the brain.
- Somatic nervous system
- A part of the peripheral nervous system that uses cranial and spinal nerves in volitional actions.
- Spina bifida
- A developmental disease of the spinal cord, where the neural tube does not close caudally.
- Sulcus
- (plural form, sulci) The crevices or fissures formed by convolutions in the brain.
- Sympathetic nervous system
- A division of the autonomic nervous system, that is faster than its counterpart that is the parasympathetic nervous system and works in opposition to it. Generally engaged in “fight or flight” functions.
- Temporal lobe
- An area of the cerebrum that lies below the lateral sulcus; it contains auditory and olfactory (smell) projection regions.
- Thalamus
- A part of the diencephalon that works as a gateway for incoming and outgoing information.
- Transduction
- A process in which physical energy converts into neural energy.
- Wernicke’s area
- A language area in the temporal lobe where linguistic information is comprehended (Also see Broca’s area).
- White matter
- Regions of the nervous system that represent the axons of the nerve cells; whitish in color because of myelination of the nerve cells.
- Working memory
- Short transitory memory processed in the hippocampus.
References
- American Association for the Advancement of Science (AAAS). (1880). Dr. Paul Broca. Science, 1(8), 93. http://www.jstor.org/stable/2900242
- Bullock, T. H. (1984). Comparative neuroscience holds promise for quiet revolutions. Science, 225(4661), 473–478.
- Crick, F., & Koch, C. (1990). Towards a neurobiological theory of consciousness. Seminars in the Neurosciences, 2, 263–275.
- Darwin, C. (1871). The descent of man, and the selection in relation to sex. London: J. Murray.
- Darwin, C. (1859). On the origins of species by means of natural selection, or, The preservation of favoured races in the struggle for life. London, UK: J. Murray.
- Demonet, J. F., Chollet, F., Ramsay, S., Cardebat, D., Nespoulous, J. L., Wise, R., . . . Frackowiak, R. (1992). The anatomy of phonological and semantic processing in normal subjects. Brain, 115(6), 1753–1768.
- Edelman, G. (2004). Wider than the sky: The phenomenal gift of consciousness. New Haven, CT: Yale University Press.
- Gardner, R. A., & Gardner, B. T. (1969). Teaching sign language to a chimpanzee. Science, 165(3894), 664–672.
- Goodall, J. (1986). The chimpanzees of Gombe: Patterns of behavior. Cambridge, MA: Harvard University Press.
- Hubel, D. H. (1995). Eye, brain, and vision. Freeman & Co., NY: Scientific American Library/Scientific American Books.
- Kandel, E. R., & Schwartz, J. H. (1982). Molecular biology of learning: Modulation of transmitter release. Science, 218(4571), 433–443.
- Knapp, M. L., & Hall, J. A. (2009). Nonverbal communication in human interaction. Boston, MA: Wadsworth Cengage Learning.
- Ramón y Cajal, S. (1937). Recollections of my life. Memoirs of the American Philosophical Society, 8, 1901–1917.
- Rolls, E. T., & Cowey, A. (1970). Topography of the retina and striate cortex and its relationship to visual acuity in rhesus monkeys and squirrel monkeys. Experimental Brain Research, 10(3), 298–310.
- Rosenzweig, M. R., Breedlove, S. M., & Leiman, A. L. (2002). Biological psychology (3rd ed.). Sunderland, MA: Sinauer Associates.
- Smith, E. E., & Jonides, J. (1999). Storage and executive processes in the frontal lobes. Science, 283(5408), 1657–1661.
- Smith, E. E., & Jonides, J. (1997). Working memory: A view from neuroimaging. Cognitive psychology, 33, 5–42.
- Van Essen, D. C., Anderson, C. H., & Felleman, D. J. (1992). Information processing in the primate visual system: An integrated systems perspective. Science, 255(5043), 419–423.
- Walker, L. A. (1987). A loss for words: The story of deafness in a family. New York, NY: Harper Perennial.
How to cite this Chapter using APA Style:
Ahmad, A. (2019). The nervous system. Adapted for use by Queen’s University. Original chapter in R. Biswas-Diener & E. Diener (Eds), Noba textbook series: Psychology.Champaign, IL: DEF publishers. Retrieved from http://noba.to/wnf72q34
Copyright and Acknowledgment:
This material is licensed under the Creative Commons Attribution-NonCommercial-ShareAlike 4.0 International License. To view a copy of this license, visit: http://creativecommons.org/licenses/by-nc-sa/4.0/deed.en_US.
This material is attributed to the Diener Education Fund (copyright © 2018) and can be accessed via this link: http://noba.to/wnf72q34.
Additional information about the Diener Education Fund (DEF) can be accessed here.
Ramón y Cajal, S. (1937). Recollections of my life. Memoirs of the American Philosophical Society, 8, 1901–1917.
Crick, F., & Koch, C. (1990). Towards a neurobiological theory of consciousness. Seminars in the Neurosciences, 2, 263–275.
Edelman, G. (2004). Wider than the sky: The phenomenal gift of consciousness. New Haven, CT: Yale University Press.
Gardner, R. A., & Gardner, B. T. (1969). Teaching sign language to a chimpanzee. Science, 165(3894), 664–672.
Goodall, J. (1986). The chimpanzees of Gombe: Patterns of behavior. Cambridge, MA: Harvard University Press.
Knapp, M. L., & Hall, J. A. (2009). Nonverbal communication in human interaction. Boston, MA: Wadsworth Cengage Learning.
Kandel, E. R., & Schwartz, J. H. (1982). Molecular biology of learning: Modulation of transmitter release. Science, 218(4571), 433–443.
Bullock, T. H. (1984). Comparative neuroscience holds promise for quiet revolutions. Science, 225(4661), 473–478.
Walker, L. A. (1987). A loss for words: The story of deafness in a family. New York, NY: Harper Perennial.
A human ancestor, handy man, that lived two million years ago.
Modern man, the only surviving form of the genus Homo.
Darwin, C. (1859). On the origins of species by means of natural selection, or, The preservation of favoured races in the struggle for life. London, UK: J. Murray.
Darwin, C. (1871). The descent of man, and the selection in relation to sex. London: J. Murray.
Rosenzweig, M. R., Breedlove, S. M., & Leiman, A. L. (2002). Biological psychology (3rd ed.). Sunderland, MA: Sinauer Associates.
The outermost layer of a developing fetus.
A process that causes the formation of the neural tube.
A front-back plane used to identify anatomical structures in the body and the brain.
A developmental disease of the spinal cord, where the neural tube does not close caudally.
A part of the nervous system that contains the cerebral hemispheres, thalamus, and hypothalamus.
Brain progenitor cells that asymmetrically divide into other neuroblasts or nerve cells.
The lining of the neural tube.
A set of primordial neurons that migrate outside the neural tube and give rise to sensory and autonomic neurons in the peripheral nervous system.
A part of the peripheral nervous system that connects to glands and smooth muscles. Consists of sympathetic and parasympathetic divisions.
A part of the peripheral nervous system that uses cranial and spinal nerves in volitional actions.
A division of the autonomic nervous system that is slower than its counterpart—that is, the sympathetic nervous system—and works in opposition to it. Generally engaged in “rest and digest” functions.
A division of the autonomic nervous system, that is faster than its counterpart that is the parasympathetic nervous system and works in opposition to it. Generally engaged in “fight or flight” functions.
Consists of left and right hemispheres that sit at the top of the nervous system and engages in a variety of higher-order functions.
(plural form, sulci) The crevices or fissures formed by convolutions in the brain.
(plural form, gyri) A bulge that is raised between or among fissures of the convoluted brain.
The major fissure that divides the frontal and the parietal lobes.
The major fissure that delineates the temporal lobe below the frontal and the parietal lobes.
An area of the cerebrum that lies below the lateral sulcus; it contains auditory and olfactory (smell) projection regions.
A strip of cortex just in front of the central sulcus that is involved with motor control.
Cortical space projected by an area of sensory input (e.g., mm of cortex per degree of visual field).
Rolls, E. T., & Cowey, A. (1970). Topography of the retina and striate cortex and its relationship to visual acuity in rhesus monkeys and squirrel monkeys. Experimental Brain Research, 10(3), 298–310.
An area in the frontal lobe of the left hemisphere. Implicated in language production.
The most forward region (close to forehead) of the cerebral hemispheres.
Due to damage of the Broca’s area. An inability to produce or understand words.
American Association for the Advancement of Science (AAAS). (1880). Dr. Paul Broca. Science, 1(8), 93. http://www.jstor.org/stable/2900242
The form of memory we use to hold onto information temporarily, usually for the purposes of manipulation.
Smith, E. E., & Jonides, J. (1997). Working memory: A view from neuroimaging. Cognitive psychology, 33, 5–42.
Smith, E. E., & Jonides, J. (1999). Storage and executive processes in the frontal lobes. Science, 283(5408), 1657–1661.
An area of the cerebrum just behind the central sulcus that is engaged with somatosensory and gustatory sensation.
A strip of cerebral tissue just behind the central sulcus engaged in sensory reception of bodily sensations.
A language area in the temporal lobe where linguistic information is comprehended (Also see Broca’s area).
Demonet, J. F., Chollet, F., Ramsay, S., Cardebat, D., Nespoulous, J. L., Wise, R., . . . Frackowiak, R. (1992). The anatomy of phonological and semantic processing in normal subjects. Brain, 115(6), 1753–1768.
A fiber tract that connects Wernicke’s and Broca’s speech areas.
Due to damage of Wernicke’s area. An inability to recognize objects, words, or faces.
The back part of the cerebrum, which houses the visual areas.
A part of the diencephalon that works as a gateway for incoming and outgoing information.
Hubel, D. H. (1995). Eye, brain, and vision. Freeman & Co., NY: Scientific American Library/Scientific American Books.
A process in which physical energy converts into neural energy.
Van Essen, D. C., Anderson, C. H., & Felleman, D. J. (1992). Information processing in the primate visual system: An integrated systems perspective. Science, 255(5043), 419–423.
A loosely defined network of nuclei in the brain involved with learning and emotion.
(plural form, hippocampi) A nucleus inside (medial) the temporal lobe implicated in learning and memory.
(plural form, fornices) A nerve fiber tract that connects the hippocampus to mammillary bodies.
A medial cortical portion of the nervous tissue that is a part of the limbic system.
A nucleus of the basal ganglia.
Part of the diencephalon. Regulates biological drives with pituitary gland.
A bridge that connects the cerebral cortex with the medulla, and reciprocally transfers information back and forth between the brain and the spinal cord.
An area just above the spinal cord that processes breathing, digestion, heart and blood vessel function, swallowing, and sneezing.
A nervous system structure behind and below the cerebrum. Controls motor movement coordination, balance, equilibrium, and muscle tone.
Composes the bark or the cortex of the cerebrum and consists of the cell bodies of the neurons (see also white matter).
Regions of the nervous system that represent the axons of the nerve cells; whitish in color because of myelination of the nerve cells.
A method of staining tissue including the brain, using antibodies.
A surgical method in which a part of the animal brain is removed to study its effects on behavior or function.
A physiological measure of large electrical change in the brain produced by sensory stimulation or motor responses.
A noninvasive brain-scanning procedure that uses X-ray absorption around the head.
Or MRI is a brain imaging noninvasive technique that uses magnetic energy to generate brain images (also see fMRI).
(or PET) An invasive procedure that captures brain images with positron emissions from the brain after the individual has been injected with radio-labeled isotopes.