Chapters
1
Overview
In this chapter, we discuss some of the foundational concepts in pathophysiology: How are cells and tissues organized? How is homeostasis maintained? And how is communication and control maintained among cells, tissues, and organ systems?
Learning Outcomes
Completion of the readings and learning activities in this chapter will enable learners to:
1.1: Identify the components and functions of cellular organelles
1.2: Describe how homeostasis is maintained
1.3: Understand the mechanisms by which cells communicate
1.4: Articulate the main features of response and feedback loops
Key Concepts
• While conditions external to the cell may fluctuate within physiologic ranges, intracellular conditions must be tightly controlled.
• To maintain homeostasis, cells must communicate via several different mechanisms.
• Second messenger systems amplify extracellular signals.
• Loss of homeostasis is a basis of disease.
• Negative feedback and feedforward reflexes help to compensate for changes in the environment.
• Positive feedback loops are not homeostatic.
Learning Units
Unit 1.2 Homeostasis and Feedback Loops
Unit 1.3 Cellular Communication
Unit 1.1: Cells are the Basic Units of Life
Cells are the basic unit of life and heredity. Maintenance of health and ‘ordered’ physiology is contingent on cellular integrity and optimal function. Moreover, at some level, all diseases are the result of ‘disordered’ cellular physiology. To that end, it is helpful to review some key elements of cell structure and function. In the diagram below (Figure 1), we depict some of the main membrane-bound and non-membrane-bound components of cells.
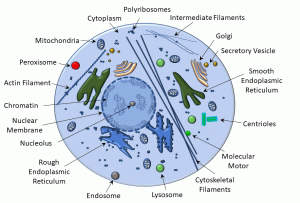
The nucleus is a double lipid bilayer, which has highly organized channels (nuclear pores) that regulate the passage of ribosomal RNA and even ions. The interior of the nucleus (‘nucleoplasm‘) contains DNA that may be condensed into either heterochromatin (condensed) or euchromatin (dispersed). The nucleolus is the site of ribosome biogenesis.
Non-membrane-bound organelles include: polyribosomes, cytoskeleton (including microtubules, intermediate filaments, and actin filaments), molecular motors, and centrioles.
The cytoplasm of the cell comprises a rich mixture of inorganic elements (electrolytes, metals, cofactors) and organic molecules (sugars, amino acids, peptides, and proteins).
Self-Study Question:
• What are the basic functions of each of the cellular components listed above?
The Plasma Membrane
The plasma membrane is a semi-permeable membrane, which means that it allows some molecules to pass from the extracellular space into the cytoplasm, and not others. The basic element is a phospholipid bilayer. The internal lipid layer is ‘lipophilic’. Non-polar molecules (eg: steroids) may easily pass through the membrane. In contrast, large molecules (eg: proteins) and charged molecules (eg: ions) can pass only by means of facilitated transport (eg: pores, carrier proteins, or ‘pumps’ that use energy). Glycolipids, glycoproteins, and lipids populate the plasma membrane, as depicted below (Figure 2).
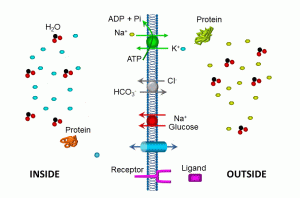
Illustrated in this diagram is a more schematic view of membrane transport molecules, including the ubiquitous sodium/potassium ATPase, a sodium/glucose symporter, a bicarbonate/chloride antiporter, an ion channel, and a plasma membrane receptor. Let’s summarize some of these transport mechanisms:
- ATPases move molecules against their concentration gradient; in the example above, three sodium (depicted in tellow) are moved out of the cell for every two potassium (depicted in blue) moved into the cell. This maintains a low intracellular sodium gradient (compared to the outside of the cell), and high potassium levels inside the cells. Such transporters utilize cellular energy in the form of adenosine triphosphate (ATP). Essentially, high-energy phosphate bonds are used to cause conformational changes in the pump protein, releasing adenosine diphosphate and phosphate molecules. If cellular ATP levels are inadequate, as in the case of hypoxic cell injury, these and other transporters fail, resulting in shifts of both water and electrolytes.
- The sodium/glucose symport utilizes the low intracellular sodium gradient to transport sodium and glucose into the cell.
- The chloride/bicarbonate antiporter exchanges these two anions (negatively charged ions) in opposite directions, thus maintaining electroneutrality. The direction of this transporter depends on the relative concentrations of these two ions. Such transport proteins do not directly require energy, depending instead on concentration gradients.
- Ion channels also do not require energy, relying instead on concentration gradients for the transmembrane passage of ions. Most ion channels are relatively selective: for example, sodium channels allow sodium to pass far more easily that other ions. This is due in part to the charge of each ion, and also to the hydrated radius.
Proper functioning of these transporter mechanisms is fundamental to cellular homeostasis, and several disease states are the result of disordered cellular transport mechanisms (eg: cystic fibrosis).
Self-Study Question:
• How do water molecules pass through the plasma membrane?
Aerobic Cellular Metabolism
Broadly defined, metabolism is the sum of biochemical processes that occur within cells. There are different ways to further parse this term: anabolism refers to processes leading to the formation of biomolecules, whereas catabolism refers to the breakdown of organic substances. In terms of cellular energetics, we will refer below to metabolic pathways in which some food substrates are turned into energy. As depicted below, the process of aerobic metabolism begins with the intake of substrates such as glucose, and involve pathways in the cytoplasm, the matrix of mitochondria, and the inner mitochondrial membrane (Figure 4).
Figure 4: Cellular aerobic metabolism
Cellular food substrates can include sugars, fats, and proteins. Sugars in particular are high-yield substrates, given the energy stored in covalent carbon-carbon bonds. The actual energy-yielding processes may be aerobic (requiring oxygen) or anaerobic (not requiring oxygen). Aerobic metabolism is by far more efficient, and we can summarize the major biochemical steps as follows:
- Once glucose enters the cell, carbon-carbon bonds are broken during a process known as glycolysis. This occurs via the action of enzymes in the cytoplasm, yielding ATP, a 3-carbon molecule called pyruvate, and nicotinamide adenine dinucleotide (NAD), which picks up a hydrogen from glucose, yielding NADH.
- Pyruvate enters another set of reactions that occur within the inner space or ‘matrix’ of mitochondria, called the trichloroacetic acid (TCA) cycle. This process also yields ATP, as well as carbon dioxide and NADH, the latter entering into the electron transport chain.
- The electron transport chain is a process that takes place within the inner mitochondrial membrane. Essentially, this consists of a series of molecules which transfer electrons from electron donors to electron receptors though a series of oxidation and reduction reactions. A handy acronym is ‘LEO says GER’: Lose Electron – Oxidation; Gain Electron – Reduction.
- This transfer of electrons is coupled to the exchange of protons (hydrogen ions) which ultimately drives a molecule called ATP synthase, which produces ATP.
- The electron transport chain has an absolute requirement for oxygen.
A key point is that ATP is required for a variety of cellular processes, including ‘powering’ the sodium/potassium ATPase and muscle contraction, to name a couple. A lack of ATP or energy is harmful to cells. Essentially, hypoxia, or low tissue oxygen, deprives the cell of oxygen needed for electron transport, and consequently the major source of cellular ATP. Anaerobic metabolism also occurs within the cytoplasm of all cells and, while producing some ATP, is limited and results in the production of metabolic acids such as lactic acid.
Self-Study Question
• What are some of the by-products of sustained anaerobic metabolism?
Organization of Cells into Tissues
Thus far, we’ve depicted cells as round, indistinguishable structures. Of course, there are a variety of cell shapes and specializations that are adapted to specific functions. We can group collections of cells into four main tissue types (Figure 5):
Figure 5: Cell and tissue types
Epithelia:
• Epithelia are characterized by cells which are tightly packed; a basement membrane comprised of proteins such as collagen; a free surface; and a lack of vascularity.
• Subtypes of epithelia may be classified according to number of layers (simple = single layer of cells, all touching a basement membrane; stratified = multiple layers; pseudostratified = a single layer with cells of different heights), or based on cell shape (squamous = flat; cuboidal; and columnar)
• All epithelia rest on a basement membrane, which is a type of connective tissue (discussed below).
Connective Tissues:
• In this type of tissue, cells are separated by a watery matrix which may include organic and inorganic elements, as well as fibrous proteins.
• Fibrous proteins (such as collagen and elastin) may be loosely or tightly packed, and in regular or irregular arrays.
• Bone and blood also fall into this category.
Muscle Tissue:
• Muscle cells are adapted to contract, by virtue of the internal arrangement of contractile proteins such as actin and myosin.
• Skeletal and cardiac muscle fibers have visible striations as a result of the arrangement of these contractile proteins. Skeletal muscle cells are long, unbranching, and contain multiple nuclei in a single cytoplasmic compartment, an arrangement called a syncytium. Cardiac muscle cells are often branched, have one or more nuclei, and join to one another via specialized structures called intercalated discs.
• Smooth muscle fibers are fusiform (tapered) and do not have visible striations, but do have contractile proteins.
• Individual muscle cells and groups of muscle cells are invested in connective tissues, and must be vascularized.
Nervous Tissue:
• Nervous tissues are comprised of excitable cells called neurons (shown above in a variety of shapes) as well as glial and ependymal cells.
• As with muscle tissues, nervous tissues must be vascularized, and at some level are enveloped in connective tissues.
Organs, Organ Axes
In turn, organs and organ systems are typically comprised of one or more tissue types. For example, the ‘tubular’ portion of the gastrointestinal system has an inner epithelium called the mucosa, a specialized connective tissue layer below that called the submucosa, a smooth muscle layer called the muscularis, an enteric nervous system, and another outer connective tissue layer called the adventitia. Often, functional inter-relationships between different organ systems are referred to as an ‘axis’ (eg: the gut-brain axis).
Self-Study Question
What other organ axes can you identify?
Unit 1.2: Homeostasis and Feedback Loops
From the Greek word for ‘same’ or ‘steady’, homeostasis refers to the maintenance of steady internal (to the body) physical and chemical conditions. With most physiological parameters, there is a ‘set point’ around which there is a normal range of variation that is optimal for survival (Figure 6).
Figure 6: Homeostasis
Here are a few examples:
- plasma pH (7.35–7.45)
- ion concentrations (sodium = 135–145 milliequivalents (or mEq))
- core body temperature (36.1–37.2 °C)
- fasting blood glucose (80–100 mg/dL)
- plasma triglycerides (0–150 mg/dL)
Organ and body systems compensate to resist changes away from the set point. When compensatory mechanisms are successful, homeostasis is maintained. When compensation fails, homeostasis is lost (Figure 7).
Figure 7: Homeostasis and compensation
In the example above, increased blood hydrostatic pressure, as measured by mean arterial pressure (MAP), imposes an increased force against the muscle of the left ventricle, resulting in a momentary decrease in cardiac output (the amount of blood pumped out of the heart with each beat) and tissue perfusion (blood supply to cells and tissues).
- Increased MAP stimulates baroreceptors in the aortic arch, which in turn…
- trigger compensatory responses, including activation of the sympathetic nervous system.
- Increased levels of sympathetic neurohormones (epinephrine and norepinephrine) increase the rate and force of contraction.
- This increases cardiac output and tissue perfusion, and restores homeostasis.
In this example, in patients suffering from congestive heart failure, the cardiac pump loses the ability to adequately meet the metabolic needs of the body. Compensatory mechanisms fail, and pathology results.
Feedback Loops
Regulation of homeostasis depends on control systems or reflexes. These are control pathways that are extrinsic to tissue and/or organ systems, and are either response loops or feedback loops. A response loop is the simplest form of control pathway, and is evident in many organ axes (Figure 8):
Figure 8: Response loop
One example is that of a painful stimulus when touching a sharp object with your hand. The input signal is pain, which goes to an integration center (in this case, the spinal cord), which produces an output signal – pain going to higher brain centers.
In contrast, feedback loops involve ‘rerouting’ the output signal back into either the input signal or the integrating center, thereby modifying the output signal or response. There are three main types of feedback modes: positive feedback, negative feedback, and feedforward. The example below depicts a positive feedback loop (Figure 9).
Figure 9: Positive feedback loop
The input signal is food coming into the stomach (either in the form of distension of the stomach or the presence of peptides). Two cells – chief and parietal cells – serve as the integration centers. The output signals are pepsinogen and hydrochloric acid (HCl), respectively. The acidity generated by the hydrochloric acid cleaves the precursor pepsinogen into pepsin, a digestive enzyme. The positive feedback part of this response loop is in the effect of pepsin, which causes the integration center – the chief cell – to produce more pepsin. It is important to note that, in and of itself, this positive feedback loop is NOT homeostatic. In contrast, a negative feedback loop IS a homeostatic mechanism. In the diagram below, we depict a slightly more complex system of response loops which, ultimately, feeds back into the integrating centres (this time, cells of the pituitary gland and the hypothalamus) (Figure 10).
Figure 10: Negative feedback loop
In this example, stress (either physiological or psychological) serves as the initial input signal. Integrating centers include cells in the hypothalamus, anterior pituitary, adrenal cortex, and target cells throughout the body. The response is, broadly speaking, an increase in glucose metabolism caused by the steroid hormone cortisol (which is a lipophilic ligand able to cross cell membranes). However, we can also say that CRH (corticotropin-releasing hormone) and ACTH (adrenocorticotropic hormone) are output signals. The negative feedback part of this response loop comes in the form of the effect of one of the output signals – cortisol – ‘feeding back’ into two of the integrating centers (endocrine cells of the hypothalamus and anterior pituitary gland), during which cortisol reduces the secretion of CRH and ACTH.
This reflex illustrates multiple organ and tissue axes in a homeostatic mechanism that is a fundamental part of the ‘stress response’. In this case, cortisol limits its own secretion. There is much more we could discuss relating to the stress response, although we will limit this by saying that this type of stress can be either physiological or pathological, depending on whether it facilitates the maintenance of homeostasis.
Finally, a feedforward reflex is also homeostatic in that it is anticipatory, allowing the body to anticipate a change and prevent an exaggerated response. The example depicted below illustrates the effects of ice chips when taken orally in a patient who is NPO (nothing per os or by mouth) (Figure 11).
Figure 11: Feedforward feedback
In the reflex depicted above, the initial stimulus is an increase in plasma osmolarity. The first integrating center is the hypothalamus, which has osmoreceptor cells. A second integrating center is the posterior pituitary. Output responses include antidiuretic hormone (ADH) release, as well as the increased sensation of thirst. ADH prevents fluid loss at the kidney, and the increased sense of thirst results in the intake of water, both of which will help maintain homeostasis with respect to plasma osmolarity. However, this can result in wide swings in plasma osmolarity, as it will take time for ingested water to be absorbed, and the renal effects and thirst sensation will continue the retention and ingestion of water, respectively. The feedforward or anticipatory element is seen in the effect of water (or ice) in the mouth: this triggers a decrease in thirst and reduction in ADH secretion. In this manner, a person will stop retaining water and drinking before fluid is actually absorbed, preventing fluid overloading and decreased plasma osmolarity. We have skipped a few steps along the way in this example, and it is unclear exactly what receptors may be involved in the mouth.
Self-Study Question
Elderly persons often have a decreased sense of thirst. What effects might this have on the feedback loop described above?
Unit 1.3 Cellular Communication
Modes of Communication
Inherent in all feedback loops is the ability for cells within tissues and organs to be able to communicate. Thus, cell communication is also a critical element in homeostasis. To that end, short- and long-distance modes of communication facilitate homeostatic mechanisms. This diagram depicts different mechanisms of short-distance communication (Figure 12):
Figure 12: Modes of short-distance communication
In the first example (I), neighbouring cells are depicted transmitting paracrine and autocrine signals. Paracrine signals involve messages transmitted between neighbouring cells. One example: when cells are infected by viruses, they may release an antiviral molecule, interferon gamma, which confers resistance to viral infection in neighbouring cells. Cells are able to stimulate themselves via autocrine signals: under optimal growth conditions, epithelial cells can release epithelial growth factor (EGF), which can induce growth and differentiation. Other short-distance forms of communication include direct signaling via a direct link between neighbouring cells (II). An example that we will cover in Chapter 3 involves cytotoxic T cells, which employ surface-bound proteins to link with cells that are targeted for destruction. Finally, many different types of cells share links between neighbouring cells via gap junctions (III). These are hexameric transmembrane proteins called ‘connexons’ expressed by cells that form a hollow core. These in turn link with connexons in adjacent cells, forming a continuous link between the cytoplasm of both cells. Small molecules such as ions and metabolites may transfer between these cells, enabling electrical and metabolic linkages. Gap junctions can link cardiac and smooth muscle cells, neurons, and germ cells.
Communication between cells over longer distance from a microscopic perspective is important for tissues and organ systems to coordinate their activities. For example, a cell in the anterior pituitary gland may be on the order of 10–100 micrometres (microns); a micrometre is 1/1,000,000 of a metre. In comparison, a human may be 2 metres tall; a growth signal from this cell may reach bone cells in the foot, a distance millions of times greater than the cell’s own diameter (Figure 13).
Figure 13: Modes of long-distance communication
In the upper panel (I), an endocrine-secreting cell is depicted releasing a signaling molecule into a vessel that is part of the vascular system. Target cells may be in almost any part of the body. In contrast, the nervous system sends signals between neurons and effector cells and tissues by means of electrochemical mechanisms (II). Cellular processes called axons undergo waves of depolarization which, at the axon terminal, results in the release of neurotransmitters. Finally, a combination of endocrine and neurochemical signaling is depicted in the last panel (III). The hypothalamic-pituitary axis is an example of this type of system, as depicted in the previous example of negative feedback.
Ligands and Receptors
Thus far, we have not described ‘what happens next’ – that is, what occurs when signalling molecules reach target cells. Most if not all signalling molecules bind to receptors, which we have discussed in Unit 1.1. As was also mentioned in that unit, a molecule which binds to a receptor is called a ligand. However, a further ‘wrinkle’ is that there are different types of ligands and different type of receptors. To begin, we can distinguish between two types of ligands: lipophobic and lipophilic (Figure 14).
Figure 14: Lipophobic and lipophilic ligands
Lipophilic signals cross the plasma membrane. They are able to do so because of the structure of the plasma membrane: it is a lipid bilayer (Unit 1.1), and the chemistry of these signals makes them miscible (dissolvable) within the bilayer. Examples of lipophilic ligands include steroid hormones (testosterone, estrogen, aldosterone), which have intracellular receptors. In contrast, lipophobic signals do not cross the membrane. These are typically charged molecules which cannot pass through the lipid bilayer. Most ligands are lipophobic, and therefore require extracellular receptors. One generalization about lipophilic ligands is that they typically activate receptors which function as ‘transcription factors’ – molecules which activate specific genes in the nucleus, resulting in gene products such as RNA and proteins. As a result, lipophilic ligands do not typically produce immediate responses from the cells they target. We will NOT discuss these types of receptor-ligand reactions further, except for a brief reference in Unit 4.
Self-Study Question:
We will, however, go into more depth about lipophobic ligand-receptor interactions that occur on the cell surface. When a ligand binds to a receptor, a receptor-ligand complex forms, resulting in a change in conformation of the receptor. This usually (though not always) activates a cellular process. While there is a tremendous diversity in signalling pathways, we can group these into three general modes: (i) receptors linked to ion channels; (ii) kinase– and enzyme-linked receptors; and (iii) G protein-coupled receptors (Figure 15).
Figure 15: Voltage- and ligand-gated ion channels
In the example above, a receptor is attached to an ion channel. For example, some sodium ion channels have receptors for acetylcholine. Neurohormones such as serotonin, GABA, and glutamate also bind to different ion channels in the central nervous system. These are referred to as ligand-gated ion channels (gating refers to opening or closing a channel). Once a ligand binds to the receptor site either on or near the ion channel, a conformational shift (or shape change) occurs in some of the channel proteins, allowing ions to flow down their chemical gradients.
Also depicted above is a voltage-gated ion channel. In this case, a change in the electropotential difference (transmembrane voltage) across the plasma membrane causes a conformation change in channel proteins, causing them to open and ions to flow in or out. These channels are features of excitable cells, such as neurons. It is beyond the scope of this short overview to discuss in detail how transmembrane voltages are generated and how neurons use these voltage gradients to signal distant cells; as a brief generalization, neurons are able to utilize changes in membrane potential to release ligands called neurotransmitters.
Another class of receptors are those which are kinase- and enzyme-linked (Figure 16).
Figure 16: Kinase- and enzyme-linked receptors
In these types of ligand-receptor interactions, once the ligand forms a complex with its receptor, a kinase or other enzyme gets activated. There are a variety of different kinases,, but they share one thing in common: they phosphorylate intracellular proteins, which in turn alters the activity of proteins, thereby altering cellular activity.
Finally, the most numerous and diverse group of receptors are ‘G-protein coupled’. ‘G’ is an abbreviation of guanine nucleotide. In these type of ligand-receptor interactions, once the ligand-receptor complex forms, the G-protein acts as a ’molecular switch’ that may interact with other types of molecules to transmit signals into the interior of a cell (a process called ‘transduction’).
In the example below, the receptor-ligand complex interacts with its G-protein, which in turn activates an ‘amplifying enzyme’ (such as adenylyl cyclase). Note: G-proteins can be either stimulatory or inhibitory. Stimulatory G-proteins activate receptor-driven processes, while inhibitory G-proteins will prevent activation of molecular processes (Figure 17).
Figure 17: G-protein-linked receptors
It bears repeating that lipophobic ligands are transduced, meaning that their signals are transmitted through cells to produce an effect. Again, in most cases, this involves a kinase causing the phosphorylation of another protein. But consider an example we alluded to in Unit 1.2, involving endocrine signalling. In most cases, very minute quantities of a hormone are released from the endocrine cells, and they are many times diluted in body fluids, resulting in low concentrations of the hormone at the target organ. The likelihood of very many receptor-ligand complexes forming is small. How is it that signals such as hormones are able to be transduced so widely, affecting many cells? The answer, in part, lies in the amplification of lipophobic signals via molecules called second messengers (Figure 18).
Figure 18: Second messengers
In the scheme depicted above, amplifying enzymes such as adenylate cyclase induce a growing cascade of intracellular second messengers. The activation of a first-order second messenger can stimulate two or more second-order second messengers, and so on. In this fashion, even one receptor-ligand complex can have a relatively large effect.
In general, we can categorize these second messenger systems as accomplishing two effects:
- Trigger increases in intracellular calcium
- Calcium binds to calcium-binding proteins
- eg: calmodulin, calcineurin, troponin
- This regulates a vast array of cellular processes
- Calcium binds to calcium-binding proteins
- Activate protein kinases
-
- Kinases phosphorylate proteins
- This changes their shape (and therefore activity)
- Protein phosphatases antagonize (work against) kinases
It will likely come as no surprise that calcium levels and kinase activity must be tightly regulated to maintain homeostasis.
Altered Cell Signaling and Loss of Homeostasis
Before progressing into Chapter 2 (Cell and Tissue Adaptation, Injury, and Death), it is appropriate to ask a ‘so what’ question: namely, how do the previous units relate to the study of pathophysiology? This is indeed a fair question. To reassure you that this is more than esoteric, we can begin by reiterating a few points. First, to maintain homeostasis (and therefore health), cells must be able to utilize feedback loops, which in turn depends on being able to communicate effectively. Second, one doesn’t have to look very far to see many pathophysiological conditions which are the result of altered cell signaling pathways. Below is a sampling of just a few conditions which are the result of altered or disordered cell signaling:
- Whooping cough
- Bordatella pertussis is a bacterium that produces a toxin which blocks adenylate cyclase in lung epithelial cells.
- Cholera
- A toxin produced by Vibrio cholera (also a bacterium) blocks activity of G-protein gut epithelium cells.
- Diabetes Insipidus
- This is usually the result of a defective vasopressin receptor in the kidney.
- Pituitary and thyroid cancers
- Some variants are the result of the gsp oncogene, which is a defective G-protein
CHAPTER SUMMARY
In Chapter 1, we have endeavoured to review fundamental concepts of cellular structure, function, and communication. A mastery of these details is important as we go forward to discuss more examples of disordered physiology, or pathophysiology. In Chapter 2, we will build on what we have learned to discuss how cells adapt to changes in the environment, as well as consider what happens when adaptation is unsuccessful.
intracellular membranous organelle (ER). Rough ER is the site of protein synthesis. Smooth ER is the site of fat and steroid metabolism.
packages secreting proteins into vesicles
membranous intracellular organelle; site of energy production and calcium storage
double-membrane intracellular organelle; location of genetic material
small membranous intracellular vacuole containing various hydrolases involved in protein degradation
membrane-bound intracellular compartment that is part of the endocytotic pathway
membrane-bound intracellular organelle; generates peroxides that are involved in lipid metabolism and bacterial killing
non-coding RNA that is a component of ribosomes
a type of protoplasm contained within the nucleus; includes chromosomes as well as nucleotides, enzymes, and cofactors
tightly-packed DNA, coiled around histone proteins
lightly-packed chromatin that is enriched in genes
clusters of ribosomes attached to a single strand of RNA
polymers of tubulin proteins that form part of the cytoskeleton; help maintain cell structure and are involved in intracellular transport
component of the cytoskeleton; form a fibrous network within cells
components of the cytoskeleton; composed of polymers of actin, and interact with numerous other intracellular proteins
proteins which translocate along the cytoskeleton, utilizing energy to move other molecules. Examples include dynein and kinesin, which are involved in neuronal transport.
nonmenranous intracellular organelles composed of tubulin; serves as an organizing center for spindle fibres which attach to the centromere of chromosomes during cell division
lipids with an attached carbohydrate; help maintain stability of cell membrane
proteins with attached oligosaccharides
class of non-polar molecules including fats, waxes, sterols, and lipid-soluble proteins
electrogenic enzyme which utilizes ATP to pump 3 sodium ions out of the cell for every 2 potassium transported into the cell
membrane-bound protein which carried two different ions in the same direction
membrane-bound protein which carries two similarly-charged molecules in opposite directions
pore-forming membrane proteins which allow specific ions to pass via concentration gradients
membrane-bound protein that binds to specific molecules called ligands
metabolic pathway that converts glucose into pyruvate and hydrogen; produces ATP
three-carbon molecule that is an intermediate in several metabolic pathways, including the TCA cycle
series of chemical reactions occurring within mitochondria; releases stored energy via oxidation of acetyl-CoA
aerobic process which occurs via electron-carrying molecules anchored on the inner mitochondrial membrane; electrons are transferred from donors to receptors via redox reactions, releasing energy
proton-transporting complex that phosphorylates ADP into ATP
process resulting in energy release which utilizes carbohydrates and fats, using nitrates as a terminal electron acceptor
product of anaerobic metabolism
main extracellular structural protein; main component of connective tissues
extracellular matrix protein that is able to coil, and uncoil; allows many tissues in the body to resume shape after stretching
multiple nuclei in a common cytoplasmic compartment
junction between cardiac cells; contains gap junction and specialized adhesion proteins
supporting cell of the nervous system
type of glial cell lining the ventricles of the brain
the amount of substance that will react with a certain number of hydrogen ions
cell of the gastric mucosa that produces pepsinogen
gastric epithelial cell which secretes hydrochloric acid and intrinsic factor
peptide produced by the hypothalamus; part of the stress response
polypeptide produced by the anterior pituitary; increases production and release of cortisol
glucocorticoid involved in the stress response; regulates metabolism and sugar homeostasis
molecule that binds to a receptor; may be a protein or lipids
shape or structure of a molecule
enzyme that catalyzes the transfer of a phosphate group to a specific molecule
guanine nucleotide-binding protein; a family of proteins that act as molecular 'switches' in cells
enzyme with key regulatory roles in all cells