16
By Gabriel Espinosa, Jack Hanlon, and Alexander Wadsworth
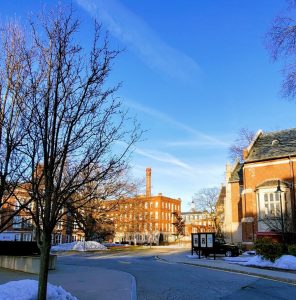
Introduction
How do we end a relationship centuries in the making? As much as we would love to be hopeful of our current climate change situation and the potential to go green in the near future, we must first face the reality of our fossil fuel based global economy. Humanity has built a world which revolves around the extraction and combustion of exhaustible fuel sources and cutting the cord, without facing the reality of our path dependence, will be catastrophic.
Although the reality of carbon lock-in, the fossil fuel-based systems path-dependent process driven by technological and institutional increasing returns to scale, paints our prolonged relationship with carbon-based energy as inevitable (Unruh, 2000). A path forward can be found with carbon capture, the ability to harness energy emissions for utilization and storage. The various technologies discussed in this paper will help to establish a transition period, allowing countries to cut back on emissions while they upgrade their energy infrastructure to renewable sources. In conjunction, a host of applications look to challenge carbon dioxide’s place in our world, bringing new modes of sequestration and reuse of destructive greenhouse gasses. As we will be discussing the feasibility of these technologies on a small scale, we decided to limit our scope of research to one specific region, as we believed this would give the best estimation of implementation and have the possibility of scaling upward. Explicitly, we will focus on the use, implementation, and application on the campus of Worcester Polytechnic Institute in Massachusetts, as they have great potential for these technologies.
Background
Carbon fuel sources were untouched by humanity up until the eighteenth century and stayed a rare commodity until the Industrial Revolution took off in the nineteenth century, once machines such as the steam engine, electric motor, and light bulb were invented. In a matter of nearly two hundred and fifty years, both the demand, production, and use of coal has increased more than two thousand-fold (Hone, 2020; Ritchie & Roser, 2017). This rapid buildup restricted flexibility among competing companies within each industry, leading all who wanted to grow throughout the economic boom straight to coal, an action which has kept all industry leaders stuck with coal to this day. As the excessive burning of fossil fuels continues, humanity has been forced into a challenging predicament: discovering a method to escape the carbon lock-in by any means possible.
Carbon lock-in is viewed as a mix of systematic forces put in place by preexisting industry that elevate fossil-based fuel sources over natural and renewable sources, despite the numerous advantages found within those other sources (Unruh, 2000). Many of the preexisting industries that managed to grow on account of these carbon filled sources continue to release exorbitant amounts of emissions, with successful attempts to bring green energy lacking. Two of the largest emissions contributors, the electricity generation and transportation industry, struggle to properly integrate these advantageous fuel sources due to an exponentially increasing demand placed upon them both (Unruh, 2000). The two come together to emit nearly 30% of all greenhouse gasses globally and in fully developed regions these levels have reached 50% of all gas output (EPA, 2020). Not all blame can be placed on current day industry though, as their infrastructure had previously been laid out for them and societies increasing demand and wealth only forces their hand toward carbon based pre-established methods (Seto et al., 2016).
Figure 2: This chart shows the total emissions produced by each major economic sector within the U.S. in 2018, showing the electricity generation and transportation sectors as the two greatest polluters. “Total U.S. greenhouse gas emissions by economic sector in 2018“, EPA (2020), United States Environmental Protection Agency, Public Domain.
As society continues to grow digitally and further rely upon technology, the need for electricity will grow along with it, just as the need for transportation will increase with the global population. The need for transportation is one of the more difficult obstacles for what is often referred to as the ‘behavioral lock-in’, a societal desire that pushes consumers in the direction of popular opinions and needs, such as owning your own car (Seto et al., 2016). The increasing number of drivers who use their own vehicles rather than shared, public transportation is often overlooked, and as more fossil fuel-based automobiles are created and registered for individuals to drive, the harder it becomes for them to be cycled out by electric cars. With electricity demands growing quickly, it has become difficult to replace existing power structures with cleaner generation methods because of our behavior and technological lock-in. These rapid advancements forced by human behavior upon those in charge of production, invention, and even research make it nearly impossible to swap fuel sources quickly and reliably. There is hope in new technology actively being developed to grab the carbon right out of our atmosphere.
Carbon capture for utilization and storage (CCUS) is the process of taking carbon out of the combustion process for industrial use or for permanent removal from the atmosphere. This technology alone has the potential to reduce carbon emissions from all sources, without having to change the source of fuel. The United Nations Environment Programme and International Energy Agency estimate that carbon capture has the potential to reduce up to 6.3 Gt of CO2 per year by 2050 (United Nations, 2014).
Industries and researchers have found multiple successful ways to incorporate this technology into current carbon producing energy sources. All CCUS technologies fall into two categories, pre-combustion capture and post-combustion capture. Pre-combustion capture is the process of separating carbon from the fuel source, leaving hydrogen to be burned for energy production (Haszeldine, 2009). Post-combustion capture is the process of absorbing carbon from the gas products of fossil fuel combustion (Haszeldine, 2009). Both processes purify carbon into CO2, which can be transported for either productive use in industry or for permanent storage.
Both types of CCUS technology have their obvious pros but share the same large con, the process of taking carbon out of fuel requires energy, and therefore costs more money to produce. Current CCUS technology for use in industrial settings has shown a 25-40% energy tax on power plants, however current carbon capture research has the energy tax dropping to 10% and capture efficiency increasing (Haszeldine, 2009). Large energy taxes such as this cause large spikes in cost of electricity, with a U.K. study reporting a cost increase of 10% per household if carbon capture technology were applied to the energy sector (Haszeldine, 2009).
Despite the current disadvantages these technologies bring, they are a gateway into a new world of renewable energy. The purpose of carbon capture is to reduce the amount of emissions from current harmful practices without having to force a large change on the industries dependent on coal and natural gas (Dwortzan, 2020). CCUS technology also provides a future where clean energy is guaranteed in all circumstances. Renewable energy is a perfect solution to green energy demands but they are not perfectly reliable. A study in the U.K. found that wind power outputs can vary up the 30%, and there are rare weeks where wind energy generation ceases (Haszeldine, 2009). While this unreliability is rare, fossil fuels can be used to generate power on a small scale with carbon capture creating a permanent emission-free future for energy.
Approach and Methodology
We have investigated the ideal combination to use on a small-scale fossil fuel power generator and heating system and are currently collaborating with professionals to work out the application process. We gathered a wide range of information regarding different applications of captured carbon as well as optimal technologies that had significant research and backing, both from larger commercial companies and government agencies. A large portion of our data was gathered through peer-reviewed journals and research papers, all found within our references. The remainder was accumulated from statistics published by government sources and commercial settings. In terms of our contacts, we approached experts both around campus and about carbon capture technology. One such contact was Dr. Eggleston, a civil and environmental engineering head at WPI, who talked us through the analysis required to implement these technologies, as well as the economics behind creating such a system. Another expert that we reached out to successfully was Mr. Spratt, the director of energy, utilities, and contracted services at the university, who divulged much needed information regarding statistics and information about the power and heat generation site. We are currently still in contact with Dr. Voskian, the creator of the electro-swing technology, about a possible joint venture between WPI, MIT, and his company Verdox. We contacted other companies around the country that deal with CCUS technology in a commercial setting to get a better idea of how operating and maintaining these systems works. We were able to research their patents and use open data to analyze the effectiveness of their solutions on their own relative scale. All this collection was done with the goal of developing a cost benefit analysis and plan for the implementation of a carbon capture system within the WPI natural gas powerhouse.
Findings and Results
Our research on carbon capture included numerous possible technologies that have been developed or are currently being researched. We concluded, based on complexity and data-supported efficiency, that the ideal carbon capture systems are the Allam Cycle and Faradaic Electro-Swing Reactive Adsorption (ESA).
The Allam cycle is a rendition of known oxy-fuel cycles that captures all atmospheric emissions from traditional carbon-based fuel sources. The system works by using the supercritical CO2 and water generated from the combustion of natural gas or coal in pure oxygen to spin a turbine for electricity generation. The gases are then funneled through a water separator, leaving only pure CO2 left in the system. Then, approximately 5% of the remaining carbon dioxide can be exported out of the system at standard CO2 piping pressure and temperature, leaving the rest to get pumped back through and reused (Allam et al., 2017).
Figure 3: Simplified schematic of commercial scale Allam cycle with a natural gas fuel source. Allam et al. 2017. CC BY-NC-ND 4.0.
This newly developed energy cycle has significant advantages over normal power plants that do not integrate carbon capture systems. It can achieve higher efficiencies, upwards of 59%, with standard energy generation which can only reach up to 50% efficiency (Allam et al., 2017). It should be reiterated that this technology is not inherently a carbon capture device, it is a power cycle that can reliably capture 100% of greenhouse gas emissions.
Faradaic Electro-Swing Reactive Adsorption is a new technology, as of 2019, developed by MIT researchers Dr. Voskian and Dr. Hatton. The system works by running carbon dioxide rich flue gas over electrochemical cells comprised of polyanthraquinone and polyvinylferrocene electrodes seen in Figure 4. The carbon dioxide can saturate the cells by creating voltage swings within the system, this captures carbon dioxide while allowing the remainder of the flue gas to pass through unimpeded (Voskian & Hatton, 2019). This process subsequently charges the cells, putting energy back into the system. Once the electrochemical cells are saturated, flue gas can be cut off and the polarity of the cells reversed. This would release the pure carbon dioxide where it can be directed to a proper containment and storage system. With current innovations in the technology, the electrodes can go through nearly 100,000 charging cycles before needing replacement. The overall efficiency of the ESA is dependent on the energy inputted, at 90kJ per mole carbon dioxide the system has a 100% capture rate (Voskian & Hatton, 2019). The primary benefit to ESA is its independence of any temperature or pressure differentials typically required in carbon capture technologies. Simply relying on the charging and discharging of electrochemical cells greatly reduces the energy demands of the process and decreases mechanical complexities.
Figure 4: Diagram modeling the electro-swing carbon capture system, image (a) displays the stacked electrochemical cells in contact with passing carbon dioxide, (b) stack of electrochemical cells. “Figure 7” by Voskian & Hatton, 2019, CC BY-NC 3.0
As for possible applications of the carbon dioxide captured by the implemented technology, there are a plethora of possibilities WPI could begin to practice. Greenhouse enrichment is a commonly used process in which CO2 is directly released within greenhouses to bolster plant growth by increasing the rate at which photosynthesis occurs. Replacing the unnatural production for this purpose diminishes levels of the harmful gas in the atmosphere and repurposes the byproduct of electricity and heat generation in a robust manner. The carbonation of professional pools is also managed using carbon dioxide, as it is able to balance the pH levels of bodies of water. WPI is an institution that employs this method to keep their indoor pool regulated by purchasing carbon dioxide gas. Piping CO2 directly captured by the technology implemented would be both a cost-effective and environmentally friendly practice, lowering their emissions rate and cutting spending on pool maintenance (Gunter et al., 1998; Gomà et al., 2010).
As previously stated, storing, and selling any byproduct of energy production is a viable and extremely cost-effective application of the captured gasses. While the resale of this gas cannot guarantee its later emission by other processes, it would offset possible production of unnecessary and harmful greenhouse gases toward our climate. As the world moves toward decarbonization of industry, the amount of CO2 able to be produced and sold will decrease, raising the prices of the gas linearly over time, generating a large source of revenue for any organization that implements CCUS technology (Luckow et al., 2015).
The WPI on-campus powerhouse is actually a misnomer, rather than generating any power, the building simply houses natural gas boilers used for campus heating. All of the University’s 28.7 million kWh of electricity used annually is sourced from the Massachusetts power grid, comprised of various renewable and nonrenewable sources (WPI, 2019). However, the burning of natural gas is still responsible for the output of carbon dioxide. With limited data available regarding the Universities energy metrics, we calculated that WPI burns 144,648,023 cubic feet of natural gas yearly for its heating demands. Using the average efficiency of natural gas boilers, we found that the campus would emit 7,432 metric tons of carbon dioxide every year from burning the previously mentioned quantity of gas (WPI, 2019).
In order to decide which technology could most reasonably be implemented on the WPI campus, we created a cost benefit analysis to breakdown the real-world investments associated with each solution. Table 1 pictured below represents the findings of this analysis, showing both the initial and long-term costs associated with the ESA technology. The costs attributed to each carbon dioxide utilization technique were also analyzed to account for all implementation costs more accurately, not depicted in the table.
Table 1: Cost benefit analysis of implementing an electro-swing adsorption system on the WPI campus and the associated expenses.
Beginning with the three different utilization methods discussed previously; given the rather simple and affordable implementation of both on-campus carbonation and greenhouse enrichment it was decided that both solutions would be viable uses of captured carbon dioxide. However, the relatively large amount of greenhouse gases generated on campus far exceeds the demands of the described solutions. In fact, just 12,201 kg of CO2 or 1.97% of WPI’s yearly emissions could be used by the greenhouse and pool, therefore the remainder of captured carbon would best be sold. Currently, the value of pure CO2 is increasing at a predictable rate with a current average sales price of $25 per ton. From selling its carbon dioxide, WPI would make more money than the energy cost to operate an ESA system by the year 2031. Profits from carbon dioxide sales would pay off the initial carbon capture cost by the year 2040 (Luckow et al., 2015).
Regarding the capture devices mentioned, the Allam Cycle would require the largest upfront cost at just over $86 million, roughly equivalent to the Smart World building currently in construction at WPI. However, in comparison to ESA, no additional yearly costs would be associated given the plant’s ability to generate electricity for campus using the current natural gas heating supply. Implementing this technology on campus would essentially cut off WPI from the grid, allowing the school to generate its own clean energy while selling the byproduct of its energy production. As is present in the following decision matrix, it is important to note the underlying costs in constructing such a large system as the Allam Cycle. These costs include the disruption of movement on campus and the requirement of a new heat generation system for the duration of the construction process.
Although less ambitious, the ESA system would cost only $5 million during the construction process and would be easily integrated within the current powerhouse flue gas piping network. The system would require a space of roughly 20 cubic meters which would easily fit within the space of the powerhouse. While WPI would still be dependent on the Massachusetts power grid for electricity needs, with ESA, the university could continue burning natural gas for heat without emitting carbon dioxide. In order to operate the ESA system, additional power is required, roughly 2 million kWh per year. Buying this electricity from the grid would cost an average of $423,000 yearly, however, given the remaining nonrenewable energy sources that makeup the Massachusetts grid, this additional energy would likely result in indirect statewide increased carbon emissions. However, with the previously mentioned sales market for pure carbon dioxide, these additional costs could be offset by the sale of campus captured CO2, essentially negating the additional yearly energy expenses associated with the operation of ESA.
To determine the best carbon capture technology for implementation on a small scale we created a decision matrix to further analyze the options each solution may bring. The following decision matrix will generate a solution for the ideal carbon capture technology to implement on campus with the idea that various carbon utilization methods would be used in tandem on a small scale such as the WPI campus.
Decision Matrix Criteria:
The following is a list of criteria that we used to further narrow our potential solutions based on both primary and secondary source material.
- Affordability: Is the solution relatively inexpensive? How will the system pay itself forward over time?
- Efficiency: How efficient is the system at either capturing carbon or using carbon dioxide? How does it affect existing system efficiencies?
- Integration: How easily can the technology be integrated with existing systems? Will it require any reconfiguration of utilities?
- Impact: What is the environmental impact of the technology or utilization method? What is the overall benefit?
- Scale: What is the size of the system and how easily can it be scaled based on demand or necessity?
- Reliability: Is the system reliable? What will maintenance look like? What are potential issues that could arise and how could they be mitigated?
Decision Matrix Weights:
The following is a list of the designated weights assigned to each of the above criteria based on the criteria’s significance to the outlined goals and objectives made by both primary and secondary sources and contacts.
- Affordability (20%): The system must be economically feasible on a small scale and pay itself off over time.
- Efficiency (20%): The system must be highly efficient at either capturing or reusing captured carbon.
- Integration (20%): The technology must be easy to integrate within existing systems and utilities.
- Impact (15%): The solution must be environmentally friendly and significantly decrease the carbon footprint of WPI.
- Scale (15%): The system must be able to fit within the WPI campus, and more specifically be implemented within the confines of the boiler room.
- Reliability (10%): The system must be low maintenance and have a relatively low number of possible problems.
Table 2. Applied Decision Matrix to Faradaic Electro-Swing Reactive Adsorption and Allam Cycle Technologies. Scores are given based on their importance above, each criterion can only give as many points as their percent importance.
Conclusions
We finally concluded that the Faradaic Electro-Swing Reactive Adsorption method had the greatest chance of success and proper implementation on campus. The scalability, lower cost, faster turnaround rate for building the device, and lower operational cost per ton of CO2 were the deciding factors in the end. The success of our recommendation has a couple different ways in which it can be measured. The most obvious measure for success is a lower CO2 emission rate on campus, as the permanent sequestration of carbon is the overall goal for both our project and mitigating climate change.
The use of this technology on campus has no potential risks because it in no way changes the dynamics of current natural gas boilers. The utilization of the captured carbon is an already safe system because carbon pipelines and transportation trucks have been used around the country for decades. All quality specifications defined by the UNC Energy Center for pipeline CO2 are met by the output from the Faradaic Electro-Swing Reactive Adsorption system.
The implementation of the ESA system on the Worcester Polytechnic Institute campus would have major benefits for the institution. With this successful carbon capture device, WPI could significantly lower its projected emissions on a very short time scale, while also creating a source of revenue that will decrease electricity costs after just a decade. As an almost carbon neutral campus, prospective students would come to the institution with passion for climate change mitigation and use its resources to spark research into global sustainability efforts.
The campus of WPI can be used as a pilot project for the implementation of this technology. With this foundation, other universities and academic institutions can look into developing the technology further or implementing other promising carbon capture technologies within their communities. This rapid improvement of CCUS technology has the potential of creating cheap and effective solutions that assist the world in quickly reducing carbon emissions.
Recommendations
Many paths can be taken to cut down on greenhouse gas emissions and begin to restore the global climate, and CCUS technology is just one such avenue that showed great potential. The conclusion derived from the research done only looked into the scope of how feasible the implementation would be at WPI and did not encompass plans to go fourth and actually change the infrastructure of campus. Other steps would need to be taken in order to successfully generate and carry out such a plan. A consultation with administrators and the president of the University would need to occur, in which approval and possible methods of funding would need to be given and discussed before the project can begin.
Once both of those have been received, other parties such as Dr. Voskian, contractors, and the WPI administrative board would have to craft a more detailed model of costs, construction plans, and a timetable on implementation to prepare for the introduction of CCUS. Contracts and research done individually by all parties would need to be drafted as well to solidify plans and ensure a successful transition.
See Appendix 4 for the infographic used by the team on Project Presentation Day.
References
Allum, R., Martin, S., Forrest, B., Fetvedt, J., Lu, X., Freed, D., Brown, W., Jr., Sasaki, T., Itoh, M., & Manning, J. (2017). Demonstration of the Allam Cycle: An update on the development status of a high efficiency supercritical carbon dioxide power process employing full carbon capture. Energy Procedia, 114, 5948-5966. https://doi.org/10.1016/j.egypro.2017.03.1731
Bui, M., S. Adjiman, C., Bardow, A., J. Anthony, E., Boston, A., Brown, S., … Dowell, N. M. (2018). Carbon capture and storage (CCS): the way forward. Energy & Environmental Science, 11, 1062-1176 .https://doi: 10.1039/C7EE02342A
Discepoli, G., Cinti, G., Desideri, U., Penchini, D., & Proietti, S. (2012). Carbon capture with molten carbonate fuel cells: Experimental tests and fuel cell performance assessment, International Journal of Greenhouse Gas Control, 9, 372-384. https://doi.org/10.1016/j.ijggc.2012.05.002
Douglas, A., Carter, R., Li, M., & Pint, C.L. (2018). Toward small-diameter carbon nanotubes synthesized from captured carbon dioxide: Critical role of catalyst coarsening, Applied Materials & Interfaces, 10, 19010-19018. https://doi.org/10.1021/acsami.8b02834
Duraccio, V., Grazia Gnoni, M., & Elia, V. (2015). Carbon capture and reuse in an industrial district: A technical and economic feasibility study. Journal of CO2 Utilization, 10, 23-29. https://doi.org/10.1016/j.jcou.2015.02.004
Dwortzan, M. (2020, November 18). Powering through the coming energy transition: Carbon capture and storage are key to achieving climate goals. MIT News. https://news.mit.edu/2020/powering-through-coming-energy-transition-1118
Gomà, A., Guisasola, A., Tayà, C., Baeza, J., Baeza, M., Bartrolí, A., Lafuente, & J., Bartrolí, J. (2010). Benefits of carbon dioxide as pH reducer in chlorinated indoor swimming pools. Chemosphere, 10(4), 428-435.. https://doi.org/10.1016/j.chemosphere.2010.04.064
Gunter, W. D., Wong, S., Cheel, D. B., & Sjostrom, G. (1998). Large CO2 Sinks: Their role in the mitigation of greenhouse gases from an international, national (Canadian) and provincial (Alberta) perspective. Applied Energy, 61(4), 209-227. https://doi.org/10.1016/S0306-2619(98)00042-7
He, X., & Lima, F.V. (2019). Development and implementation of advanced control strategies for power plant cycling with carbon capture. Computers & Chemical Engineering, 121, 497-509. https://doi.org/10.1016/j.compchemeng.2018.11.004
Haszeldine, R. S. (2009). Carbon capture and storeage: How green can black be? Science, 325(5948), 1647-1652. https://doi.org/10.1126/science.1172246
Hone, D. (2020, October 22). From the Industrial Revolution to Climate Change: What Happened and Why? 2041 Foundation. https://2041foundation.org/climate-change-what-happened-and-why/
House, K. Z., Baclig, A. C., Ranjan, M., van Nierop, E. A., Wilcox, J., & Herzog, H. J. (2011). Economic and energetic analysis of capturing CO2 from ambient air. PNAS, https://doi.org/10.1073/pnas.1012253108
.Keith, D., Holmes, G. St. Angelo, D., & Heidel, K. (2018). A process for capturing CO2 from the atmosphere. Joule, 2(8), 1573-1594. https://doi.org/10.1016/j.joule.2018.05.006
Kolstad, C., & Young, D. (2010). Cost analysis of carbon capture and storage for the Latrobe Valley. UC Santa Barbara Institute for Energy Efficiency, https://www.globalccsinstitute.com/archive/hub/publications/119726/cost-analysis-ccs-latrobe-valley.pdf
Liu, J., Wang, S., Zhao, B., Tong, H., & Chen, C. (2009). Absorption of Carbon Dioxide in Aqueous Ammonia. Energy Procedia, 1(1). 933-940. https://doi.org/10.1016/j.egypro.2009.01.124
Luckow, P., Stanton, E.A., Fields, S., Biewald, B., Jackson, S., Fisher, J., Wilson, R. (2015). 2015 carbon dioxide price forecast. Synapse Energy Economics, Inc. https://www.synapse-energy.com/sites/default/files/2015%20Carbon%20Dioxide%20Price%20Report.pdf
Monkman, S., MacDonald, M., Kenward, P., & Dipple, G. (2015). The effect of in-situ development of nano-calcium carbonate on industrial concrete. CarbonCure Technologies. https://parkerblock.com/pdf/divisions/bay-ready-mix/NICOM5_Monkman-and-MacDonald.pdf.
Moore, C.C.S., & Kulay, L. (2019). Effect of the implementation of carbon capture systems on the environmental, energy and economic performance of the Brazilian electricity matrix, Energies, 12(2), 331. https://doi.org/10.3390/en12020331
Newman, P. (2017). Decoupling economic growth from fossil fuels, Modern Economy, 8, 791-805. https://doi: 10.4236/me.2017.86055
Onarheim, K., Garðarsdòttir, S.Ò., Mathisen, A., Nord, L.O., & Berstad, D. (2015). Industrial implementation of carbon capture in Nordic industry sectors. NORDICCS Technical Report. https://www.sintef.no/globalassets/sintef-energi/nordiccs/d4.2.1501-d18-co2-capture-cases.pdf
Polack, R., Wood, S., & Smith, K. N. (2010). An analysis of fossil-fuel dependence in the United States with implications for community social work. Critical Social Work, 11(3), 359-375. https://doi.org/10.22329/csw.v11i3.5837
Porter, R.T.J., Fairweather, M., Kolster, C., Mac Dowell, N., Shah, N., & Woolley, R.M. (2017). Cost and performance of some carbon capture technology options for producing different quality CO2 product streams. International Journal of Greenhouse Gas Control, 57, 185-195. https://doi.org/10.1016/j.ijggc.2016.11.020
Psarras, P., He, J., Pilorgé, H., McQueen, N., Jensen-Fellows, A., Kian, K., & Wilcox, J. (2020). Cost analysis of carbon capture and sequestration from U.S. natural gas-fired power plants. Environmental Science and Technology, 54(10), 6272-6280. https://doi.org/10.1021/acs.est.9b06147
Ritchie, H., & Roser, M. (2017, October 2). Fossil Fuels. Our World in Data. https://ourworldindata.org/fossil-fuels.
Roussanaly, S. (2019). Calculating CO2 avoidance costs of carbon capture and storage for industry. Carbon Management, 10(1), 105-112. https://doi.org/10.1080/17583004.2018.1553435
Riis, F. (2018). Norway CCS demonstration project: Evaluation of Jurassic reservoirs for safe CO2 injection and storage. Fifth CO2 Geological Storage Workshop, 21-23 Nov. 2018, Utrecht, The Netherlands. https://doi.org/10.3997/2214-4609.201802954
Saboori, H., & Hemmati, R. (2016). Considering carbon capture and storage in electricity generation expansion planning. IEEE Transactions on Sustainable Energy, 7(4), 1371-1378. https://doi.org/10.1109/TSTE.2016.2547911
Scafidi, J., & Gilfillan, S. M. V. (2019). Offsetting carbon capture and storage costs with methane and geothermal energy production through reuse of a depleted hydrocarbon field coupled with a saline aquifer. International Journal of Greenhouse Gas Control, 90, 102788. https://doi.org/10.1016/j.ijggc.2019.102788
Schmelz, W.J., Hochman, G., & Miller, K.G. (2020). Total cost of carbon capture and storage implemented at a regional scale: Northeastern and midwestern United States. Interface Focus, 10, 20190065. https://doi.org/10.1098/rsfs.2019.0065
Seto, K.C., Davis, S.J., Mitchell, R.B., Stokes, E.C., Unruh, G., & Ürge-Vorsatz, D. (2016). Carbon lock-in: Types, causes, and policy implications. Annual Review of Environment and Recourses, 41, 425-452. https://doi.org/10.1146/annurev-environ-110615-085934
Sims, R. E. H., Rogner, H.-H., & Gregory, K. (2002). Carbon emission and mitigation cost comparisons between fossil fuel, nuclear and renewable energy resources for electricity generation. Energy Policy, 31(13), 1315-1326. https://doi.org/10.1016/S0301-4215(02)00192-1
Szulczewski, M. L., MacMinn, C. W., Herzog, H. J., & Juanes, R. (2012). Lifetime of carbon capture and storage as a climate-change mitigation technology. PNAS, 109(14), 5185-5189. https://doi.org/10.1073/pnas.1115347109
Tanzer, S. E., Blok, K.,& Ramírez, A. (2020). Can bioenergy with carbon capture and storage result in carbon negative steel. International Journal of Greenhouse Gas Control, 100, 103104. https://doi.org/10.1016/j.ijggc.2020.103104
Timmons, T., Harris, J., Roach, Brian. (2014). The Economics of Renewables Energy. Global Development and Environmental Institute. Tufts University, Medford, MA. http://citeseerx.ist.psu.edu/viewdoc/download?doi=10.1.1.648.183&rep=rep1&type=pdf
UNEP (2014) The Emissions Gap Report 2014.Nairobi: United Nations Environment Programme (UNEP). unenvironment.org/emissionsgap
United States Environmental Protection Agency (EPA). (2020, December 4). Sources of Greenhouse Gas Emissions. https://www.epa.gov/ghgemissions/sources-greenhouse-gas-emissions.
Unruh, G. C. 2020. Understanding carbon lock-in. Energy Policy, 28(12), 817-830. https://doi.org/10.1016/S0301-4215(00)00070-7
Voskian, S., Hatton, T.A. (2019). Faradaic electro-swing reactive adsorption for CO2 capture, Energy and Environmental Science, 12, 3530-3547. https://doi.org/10.1039/C9EE02412C
Worcester Polytechnic Institute (WPI), (2019). Campus Operations, Office of Sustainability. https://www.wpi.edu/offices/sustainability/campus-operations
The process of burning a fuel using pure oxygen, or a mixture of oxygen and flue gas, instead of air, and recycling the byproducts of the combustion to power a turbine and future combustions.
The mixture of gases resulting from combustion and other reactions in a furnace, passing off through the smoke flue, composed largely of nitrogen, carbon dioxide, carbon monoxide, methane, water vapor, and often sulfur dioxide.
A device capable of creating electrical energy from chemical reactions or using electrical energy to force specific chemical reactions.