Levels of Organization
5 Biochemistry and Metabolism
Introduction
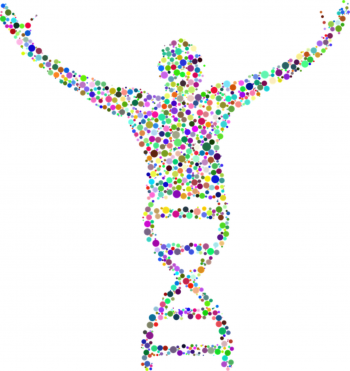
What do you think of when you hear the word “organic?”
Images of farmers’ markets and produce grown without synthetic fertilizer or pesticides? You’ll need to put those images away as you learn biology. The molecules produced by cells are organic molecules, meaning only that they contain covalently-bound carbon and hydrogen atoms.
Any large molecule is referred to as a macromolecule (macro- = “large”), and the organic compounds in this chapter all fit this description. Indeed, biological macromolecules dwarf other molecules involved in life’s chemistry, such as table salt (NaCl) or water (H2O). However, some macromolecules are made up of several “copies” of single units called monomers (mono- = “one”; -mer = “part”). Like beads in a long necklace, these monomers link by covalent bonds to form long polymers (poly- = “many”). There are many examples of monomers and polymers among organic compounds.
So, the large molecules necessary for life are built from smaller organic molecules. There are four major classes of biological macromolecules (carbohydrates, lipids, proteins, and nucleic acids), and each performs different critical functions within the cell. These molecules may also contain sulfur, phosphorus, oxygen, and nitrogen atoms, along with additional minor elements. Each molecule class has its own element “profile”, though all contain carbon, hydrogen, and oxygen. Combined, these molecules make up the majority of a cell’s mass. We will now explore the reactions cells use to break down and build up macromolecules.
Building Up and Breaking Down Macromolecules
Learning Objectives
- Compare organic and inorganic molecules.
- Identify the 4 major classes of biological macromolecules.
- Compare and contrast dehydration synthesis and hydrolysis reactions.
- Describe the role enzymes play in hydrolysis and dehydration synthesis.
Dehydration Synthesis
Most macromolecules are made from single subunits, or building blocks, called monomers. The monomers combine with each other using covalent bonds to form larger molecules known as polymers. In doing so, monomers release water molecules as byproducts. This type of reaction is called dehydration synthesis, which means “to put together while losing water.”
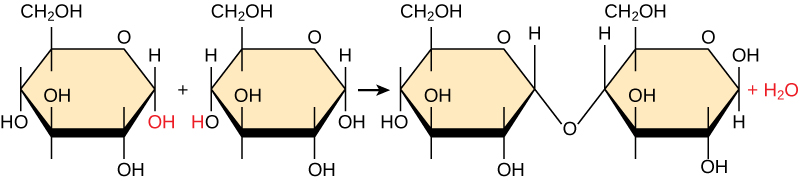
In a dehydration synthesis reaction, the hydrogen of one monomer combines with the hydroxyl group of another monomer, generating one water molecule as a byproduct of the reactant. During the same reaction, the two monomers involved share electrons and form a covalent bond between them. As additional monomers join, this chain of repeating monomers forms a polymer. Different monomer types can combine in many configurations, giving rise to a diverse group of macromolecules. Even one kind of monomer can combine in a variety of ways to form several different polymers. For example, glucose monomers are the monomers that build starch, glycogen, and cellulose.
Hydrolysis
Polymers are broken down into monomers in a process known as hydrolysis, which means “to split water.” These reactions are in contrast to dehydration synthesis (also known as condensation) reactions. Hydrolysis reactions require a water molecule to break the polymer into two parts. One part gains a hydrogen atom (H), and the other gains a hydroxyl group (-OH) from a split water molecule. This is what happens, for example, when monosaccharides are released from complex carbohydrates.
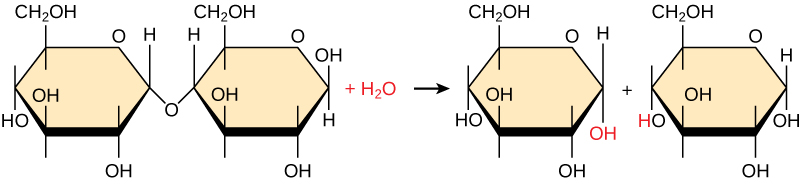
Catalyzing Dehydration Synthesis and Hydrolysis.
Dehydration and hydrolysis reactions are catalyzed, or “sped up,” by specific enzymes, a very important class of proteins. Dehydration reactions involve the formation of new bonds, requiring energy, while hydrolysis reactions break bonds and release energy. These reactions are similar for most macromolecules, but each monomer and polymer reaction is specific for its class. For example, catalytic enzymes in the digestive system hydrolyze (or break down) macromolecules in the food we eat into smaller molecules. This allows cells in our body to easily absorb nutrients (monomers) in the intestine. Each type of macromolecule is broken down by a specific enzyme. The carbohydrases break down carbohydrates. Examples include the starch amylose and enzyme amylase, the sugars sucrose, lactose, or maltose, and enzymes sucrase, lactase, or maltase. Enzymes called proteases, such as pepsin and peptidase, and hydrochloric acid break down proteins. Lipases break down lipids. Nucleases digest nucleic acids. We will discuss enzymes in more detail later in the chapter.
Learn By Doing 5.1
Compare and contrast organic and inorganic molecules.
Identify the 4 major classes of macromolecules.
Dehydration synthesis leads to the formation of
- monomers
- polymers
- water and polymers
- none of the above
During the breakdown of polymers, which of the following reactions takes place?
- hydrolysis
- dehydration
- condensation
- covalent bond
Describe the differences between dehydration synthesis and hydrolysis.
Identify the 4 broad classes of enzymes used to speed up dehydration synthesis and hydrolysis reactions.
Carbon
Learning Objectives
- Describe why carbon is well-suited to form the “backbone” of organic molecules.
- Identify organic versus inorganic molecules from chemical formulas and molecular structures.
The organic molecules made by living things are found throughout our world, in soils and seas, in commercial products, and in every cell of the human body. As mentioned, the four types critical to the structure and function of cells are carbohydrates, lipids, proteins, and nucleic acids. Before exploring these compounds, however, we first need to understand the chemistry of carbon.
It is often said that life is carbon-based. This means that carbon atoms, bonded to other carbon atoms or other elements, form the fundamental components of many, if not most, of the molecules found uniquely in living things. Other elements play important roles in biological molecules, but carbon certainly qualifies as the “foundation” element for molecules in living things. The bonding properties of carbon atoms are responsible for this important role.
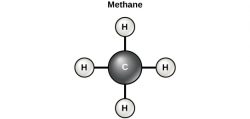
Carbon contains four electrons in its outer shell. Therefore, it can form four covalent bonds with other atoms or molecules. Recall that carbon atoms have four electrons in their valence shell and that the atoms tend to react in such a way as to complete their valence shell with eight electrons. Carbon atoms do not complete their valence shells by donating or accepting four electrons. Instead, they readily share electrons and so form covalent bonds. The simplest organic carbon molecule is methane (CH4), in which four hydrogen atoms bind to a carbon atom.
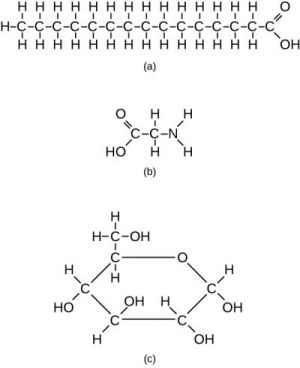
However, structures that are more complex are also made using carbon. Any of the hydrogen atoms can be replaced with a second carbon atom covalently bonded to the first. When carbon atoms share with other carbon atoms, they form a long carbon chain referred to as a carbon skeleton. In this way, long and branching chains of carbon compounds can be made, as shown in part a of Figure 5.5.
The carbon atoms may bond with atoms of other elements, such as sulfur, phosphorus, oxygen, and nitrogen (Figure 5.5., part b). The molecules may also form rings, which themselves can link with other rings (Figure 5.5., part c).
Many combinations are possible to fill the four “vacancies” in a carbon atom’s valence shell. Carbon may share electrons with oxygen, nitrogen, or other atoms in a particular region of an organic compound. Moreover, the atoms to which carbon atoms bond may also be part of a functional group. A functional group is a group of atoms linked by strong covalent bonds and tending to function in chemical reactions as a single unit. You can think of functional groups as tightly knit “cliques” whose members are unlikely to be parted. Table 5.1 shows five important functional groups in human physiology: the hydroxyl, carboxyl, amino, methyl, and phosphate groups.
Functional group | Structural formula | Importance |
---|---|---|
Hydroxyl | —O—H | Hydroxyl groups are polar. They are components of all four types of organic compounds discussed in this chapter. They are involved in dehydration synthesis and hydrolysis reactions. |
Carboxyl | O—C—OH | Carboxyl groups are found within fatty acids, amino acids, and many other acids. |
Amino | —N—H2 | Amino groups are found within amino acids, the building blocks of proteins. |
Methyl | —C—H3 | Methyl groups are found within amino acids. |
Phosphate | —P—O42– | Phosphate groups are found within phospholipids and nucleotides. |
Learn By Doing 5.2
Each carbon molecule can bond with as many as ________ other atom(s) or molecule(s).
- one
- two
- six
- four
Describe why carbon is well-suited to form the “backbone” of organic molecules.
Indicate whether the following chemical formulas represent organic or inorganic compounds.
- C6H12O6 (Glucose)
- H2O (Water)
- KI (Potassium iodide)
- MgSO4 (Magnesium sulfate)
- CH4O (Methanol)
Examine the images below. Which molecules would you classify as organic? Explain your answers in terms of the definition of an organic molecule.
Hint: Remember that you can determine the molecular formula from the diagram, though you may not need to.
Macromolecules
Learning Objectives
- Describe the basic functions of the four essential biological macromolecules.
- Identify the subunits used to build each macromolecule.
Carbon’s fondness for covalent bonding means that many distinct and relatively stable organic molecules nevertheless readily form larger, more complex molecules. As you’ve learned, monomers form polymers by dehydration synthesis. As was noted earlier, this reaction results in a polymer and water as products. Each monomer contributes: One gives up a hydrogen atom, and the other gives up a hydroxyl group. Polymers are split into monomers by hydrolysis (-lysis = “break” “rupture”). The bonds between their monomers are broken via the donation of a molecule of water, which contributes a hydrogen atom to one monomer and a hydroxyl group to the other.
Macromolecules are typically made of at least 1,000 atoms, with smaller parts of the molecule present as repeated units that are joined together. The process of polymerization links together the smaller components (monomers). The number of monomers that can be linked together leads to the large size and complexity of polymers. And it’s the large size of macromolecules that dictates their importance in living systems. They are the basis of complex cellular life. Macromolecules are not intrinsically stable. They are not created in the absence of life, nor can they persist for long outside living systems.
All cells build themselves with the four major macromolecules. They are:
- Nucleic Acids are made of nucleotide subunits linked through their phosphate backbone.
- Proteins are made of amino acid subunits linked between carbon and nitrogen.
- Lipids are typically large molecules held together with non-polar covalent bonds, making them hydrophobic. Some lipids contain covalently attached polar groups, which may act as attachment points for multiple hydrophobic lipid molecules.
- Carbohydrates have covalently linked simple sugar groups called monosaccharides.
Carbohydrates
Learning Objectives
- Describe the structure and different types of carbohydrates.
- List and explain the three major functions of carbohydrates.
The simplest of the macromolecules are carbohydrates, also called saccharides. The name “carbohydrate” is descriptive of the character of this class of molecules since they all have the general formula of a hydrated carbon: C(H2O)n. (The subscript “n” refers to how many times CH2O occurs in a particular carbohydrate.) This represents a 2:1 ratio of hydrogen to oxygen atoms (as in water), but in this case, the atoms are attached to a carbon backbone. The atoms of carbohydrates can be configured in virtually endless configurations, so carbohydrate molecules come in a multitude of different shapes and sizes.
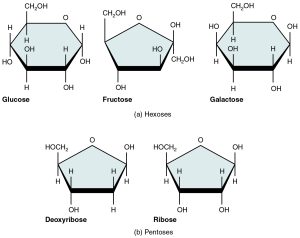
Monosaccharides (mono- = “one”; sacchar- = “sweet”) are the most basic units of carbohydrates. These are simple sugars, including glucose, fructose, and others. They contain between three and seven carbon atoms and have a sweet taste. Five monosaccharides are important in the body. Three of these are hexose sugars, so-called because they each contain six atoms of carbon. These are glucose, fructose, and galactose. The remaining monosaccharides are the two pentose sugars, each of which contains five atoms of carbon and are used by the body for energy. Carbohydrates are also critical parts of nucleotides (the monomers that our DNA is made of), with one monosaccharide, either ribose or deoxyribose, found in each. With 3 billion nucleotides present in the DNA of our cells, that is a lot of monosaccharides in the body! In the aqueous environment of the cell, monosaccharides are usually found in a ring-like structure (see Figure 5.3).
Polysaccharides are long polymers of monosaccharide sugars that are covalently bonded together. Polysaccharides are often used as a way to store the energy of monosaccharides for a limited period. These include starch (in plants) and glycogen (in animals). Polysaccharides such as cellulose are used to create the rigid structural cell wall in plants.
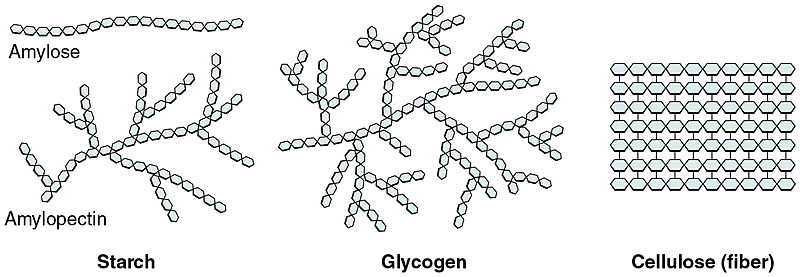
Starch is the stored form of sugars in plants and is made up of amylose and amylopectin (both polymers of glucose). Plants are able to synthesize glucose, and the excess glucose is stored as starch in different plant parts, including roots and seeds. The starch that is consumed by animals is broken down into smaller molecules, such as glucose. The cells can then absorb the glucose.
Glycogen is the storage form of glucose in humans and many other animals and is made up of monomers of glucose. Glycogen is the animal equivalent of starch and is a highly branched molecule usually stored in liver and muscle cells. Whenever glucose levels decrease, glycogen is broken down to release glucose.
Cellulose is one of the most abundant natural biopolymers. The cell walls of plants are mostly made of cellulose, which provides structural support to the cell. Wood and paper are primarily cellulose. This carbohydrate polymer is made up of glucose monomers that are linked by bonds between particular carbon atoms in the glucose molecule.
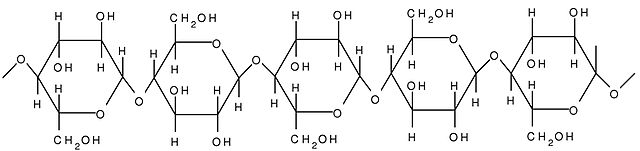
Every other glucose monomer in cellulose is flipped over and packed tightly as extended long chains. This gives cellulose its rigidity and high tensile strength, which is so important to plant cells. Cellulose passing through our digestive system is called dietary fiber. While the glucose-glucose bonds in cellulose cannot be broken down by human digestive enzymes, herbivores such as cows, buffalos, and horses are able to digest grass that is rich in cellulose and use it as a food source. In these animals, certain species of bacteria reside in the rumen (part of the digestive system of herbivores) and secrete the enzyme cellulase. The appendix also contains bacteria that break down cellulose, giving it an important role in the digestive systems of ruminants. Cellulase enzymes can break down the cellulose polymer into glucose monomers that can be used as an energy source by the animal. Thus, through differences in the structures, carbohydrates can serve the very different functions of energy storage (starch and glycogen) and structural support (cellulose).
Polysaccharides can be joined to other macromolecules. For example, complex carbohydrates can be linked with proteins or lipids to form glycoproteins and glycolipids. Very different structures can be made from a few monosaccharides arranged in different patterns and with different bonding. This flexibility in structure can therefore be used for the identification of individual cell types since the structure of each cell type is unique. More than half of the proteins in the body, which we will discuss later in this chapter, have carbohydrate modifications. The outside of cells is covered in carbohydrates attached to lipids that make up the cell membrane; we will cover lipids in the last section of this chapter.
Carbohydrates are best known as energy storage molecules, and in animals, their primary function is as a source of energy. Cells readily convert carbohydrates to usable energy. You will recall that molecules are a collection of atoms connected by covalent bonds. In general, single covalent bonds have roughly 100 units of energy associated with the force that holds the two atoms together. Table sugar, or sucrose, is the best-known carbohydrate. The most common carbohydrate in nature is glucose, which has the formula C6H12O6 and is a common energy source for many living organisms. If glucose is completely metabolized (“burned”) for its energy in a cell, it has the following chemical reaction:
C6H12O6 + 6 O2 <————-> 6 CO2 + 6 H2O and 673 units of energy
However, the body does not need dietary carbohydrates for energy, though they are a preferred fuel. Proteins and fats can meet the body’s needs, and the body can convert molecules into carbohydrates needed for energy and other cellular functions. But carbohydrates require minimal processing for use as energy. For example, a simple chemical reaction converts the disaccharide sucrose into blood sugar, which can be used directly as a source of cellular energy. The trick for the cell is to convert the 673 units of energy to a useful form so that it can do work for the cell or organism. The metabolic fate of carbohydrates will be discussed later in the course.
Another function of carbohydrates is cell recognition and signaling. This typically occurs with carbohydrates conjugated to other molecules, such as those found in glycoproteins (carbohydrates linked to proteins) and glycolipids (carbohydrates linked to lipids). Because a very large number of structures can be made from a few monosaccharides (simple carbohydrates), a very large number of different structures can also be made from a few simple carbohydrates, as will be seen later. This large number of different structures can therefore be used for the identification of individual cell types.
Carbohydrate modifications are present on lipid membranes and on proteins for specialized function and recognition. Unique carbohydrate formations allow even more specificity to a protein beyond just the amino acid code. The cell’s outer membrane is dotted with carbohydrate chains, which differ according to cell type. These carbohydrate modifications provide a “signature” of the cell and can also act as a signal. Thus, they are important in immune response and general cell-to-cell communication.
Learn By Doing 5.3
Cellulose, glycogen, and starch are examples of ________.
- monosaccharides
- disaccharides
- lipids
- polysaccharides
Cellulose, glycogen, and starch are all constructed from the same monomer. Describe how they can have different functions, given that they are all made of the same subunit.
Proteins
Learning Objectives
- Describe the four levels of protein structure and discuss the importance of protein structure in function.
- List and describe different protein functions within different cell types.
- Describe the basic structure of amino acids.
After nucleic acids such as DNA, proteins are the most important macromolecules. Structurally, proteins are the most complex macromolecules. A protein is a linear molecule made of amino acids. Just 20 different amino acids contribute to the thousands of different proteins important in human structure and function. Proteins contain a unique combination of a few dozen to a few hundred of these 20 amino acid monomers.
The sequence of a protein’s amino acids is determined by the sequence of bases in the DNA coding for the synthesis of this protein. This sequence of amino acids is a protein’s primary structure. The protein’s size, shape, and reactive properties depend on the number, type, and sequence of amino acids. The amino acid chain can remain in its primary linear structure, but often it folds up and in on itself to form a shape. This secondary structure forms from hydrogen bonding between the side chains of amino acids in the protein. These include alpha helix and beta-sheet structures. The alpha helix is dominant in hemoglobin, the protein that facilitates oxygen transport in blood. Secondary structures are integrated along with twists and kinks into a three-dimensional protein. This functional form is called the tertiary structure of the protein. An additional level of organization results when several separate proteins combine to form a protein complex—called a quaternary structure.
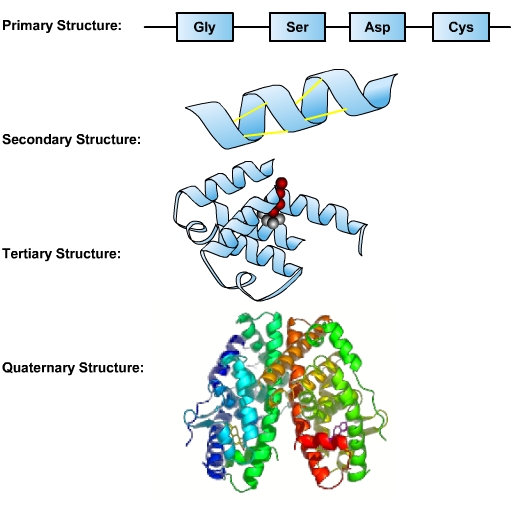
Proteins perform numerous essential functions within the cell. Many proteins serve as enzymes, which control the rate of chemical reactions, and so the responsiveness of cells to external stimuli. An enzyme can fast-forward a reaction that would take millions of years under normal conditions and make it happen in just a few milliseconds. Enzymes are important in DNA replication, transcription, and repair. Digestive processes are also largely facilitated by enzymes, which break down molecules that would otherwise be too large to be absorbed by the intestines. Enzymatic proteins also play a role in muscle contractions. The critical role enzymes play in metabolism and in making life possible will be discussed at the end of this chapter.
Other proteins are important in cell signaling and cell recognition. Some receptor proteins recognize substances as foreign and initiate an immune response. Through cell signaling, some proteins mediate cell growth and differentiation during development. Several important proteins provide mechanical support for the cell, scaffolding that helps the cell maintain its shape. Other proteins comprise much of the body’s connective tissue and structures, such as hair and nails.
Table 5.2 shows the different functional classes of proteins and provides examples of each.
Type | Functions |
Examples |
Digestive Enzymes | Help in food by catabolizing nutrients into monomeric units | Amylase, lipase, pepsin, trypsin |
Transport | Carry substances in the blood or lymph throughout the body | Hemoglobin, albumin, myoglobin |
Structural | Construct different structures, like the cytoskeleton | Actin, tubulin, keratin |
Hormones | Coordinate different body systems’ activity | Insulin, thyroxine |
Defense | Protect the body from foreign pathogens | Immunoglobulins |
Movement | allow muscle contraction | Actin, myosin |
Intercellular Communication | signal reception | Neurotransmitter receptors, self-antigens |
To build proteins, our cells need amino acids. It seems a bit inefficient, but we eat proteins, break them down into amino acids, distribute the amino acids inside the body, and then build new proteins. Our cells can synthesize some amino acids from similar ones, but essential amino acids must be obtained from the diet since they cannot be synthesized. Deficiencies of protein in the diet result in malnutrition diseases such as kwashiorkor, which is common in developing countries. In cases of kwashiorkor, protein deficiency causes edema (swelling), which leads to a distended abdomen. Proteins are eventually metabolized into ammonia and urea, which are excreted by the kidneys. Kidney disease can cause these waste products to accumulate in the body, causing someone to become very ill, ultimately leading to death. A low-protein diet can help those whose kidneys have a low level of function.
Unlike nucleic acids such as DNA, which must remain unchanged in the body for the life of the organism, proteins are meant to be transient—they are produced, do their functions, and then are recycled. The structures of proteins are also readily changed by extremes of heat or pH (due to the unfolding of the secondary and tertiary structures). When you boil an egg, the yolk and white stiffen and change color. When you cook meat, the flesh changes color and becomes firm. These changes arise because the proteins denature, changing the protein’s shape and its function.
Learn By Doing 5.4
If a protein has a quaternary structure, that means it:
- Is a small protein
- Is made of all 20 amino acids
- Is most likely coded for by multiple genes
- Has enzymatic activity
Hint: Quaternary means “fourth in rank order” and indicates the most complex version of proteins.
Different cell types express different types and concentrations of proteins. You would expect a cell common to connective tissues like ligaments and tendons to produce an abundance of proteins with which of the following functions?
- Structural
- Cell signaling
- Enzymatic
- Immune
Hint: Ligaments connect bone to bone, and tendons connect muscle to bone to maintain structural support.
Amino Acids
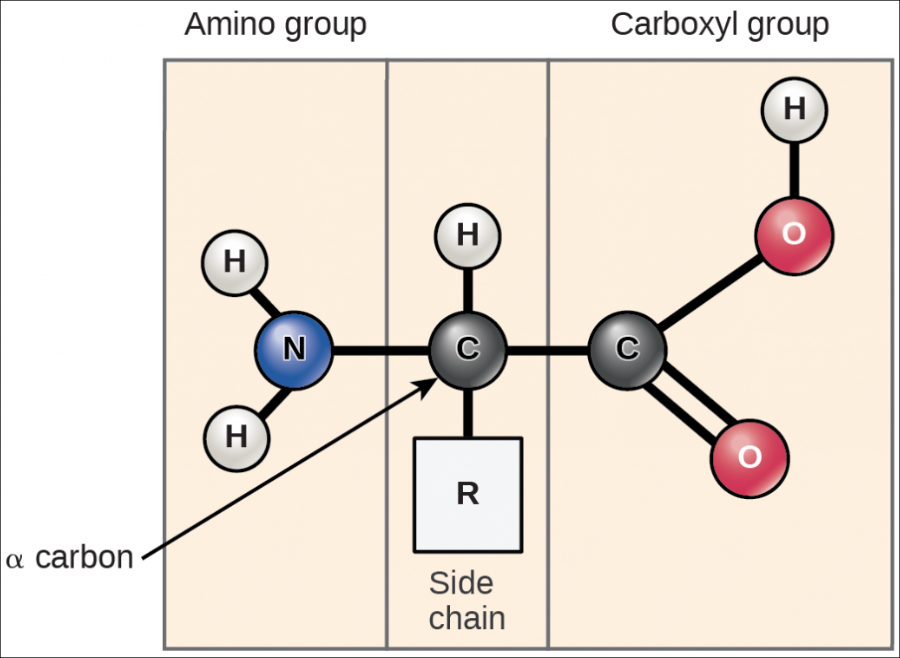
The physical and chemical properties of the 20 different naturally occurring amino acids dictate the shape of the protein and its interactions with its environment. Certain short sequences of amino acids in the protein also dictate where the protein ends up in the cell. Proteins are composed of hundreds to thousands of amino acids. As you can imagine, protein folding is a complicated process, and there are many potential shapes due to the large number of combinations of amino acids. By understanding the properties of the amino acids, you will gain an appreciation for the limits of protein folding.
All amino acids have the same backbone structure, with an amino group (the α-amino, or alpha-amino, group), a carboxyl group, an α-hydrogen, and a variety of functional groups (R) all attached to the α-carbon.
If all of the amino acids have the same basic structure with an amino, a carboxyl, and a hydrogen atom fixed to the alpha-carbon, then the large variation in the properties and structure of the amino acids must come from the fourth group attached to the alpha carbon. This group is referred to as the side chain of the amino acid or the R group.
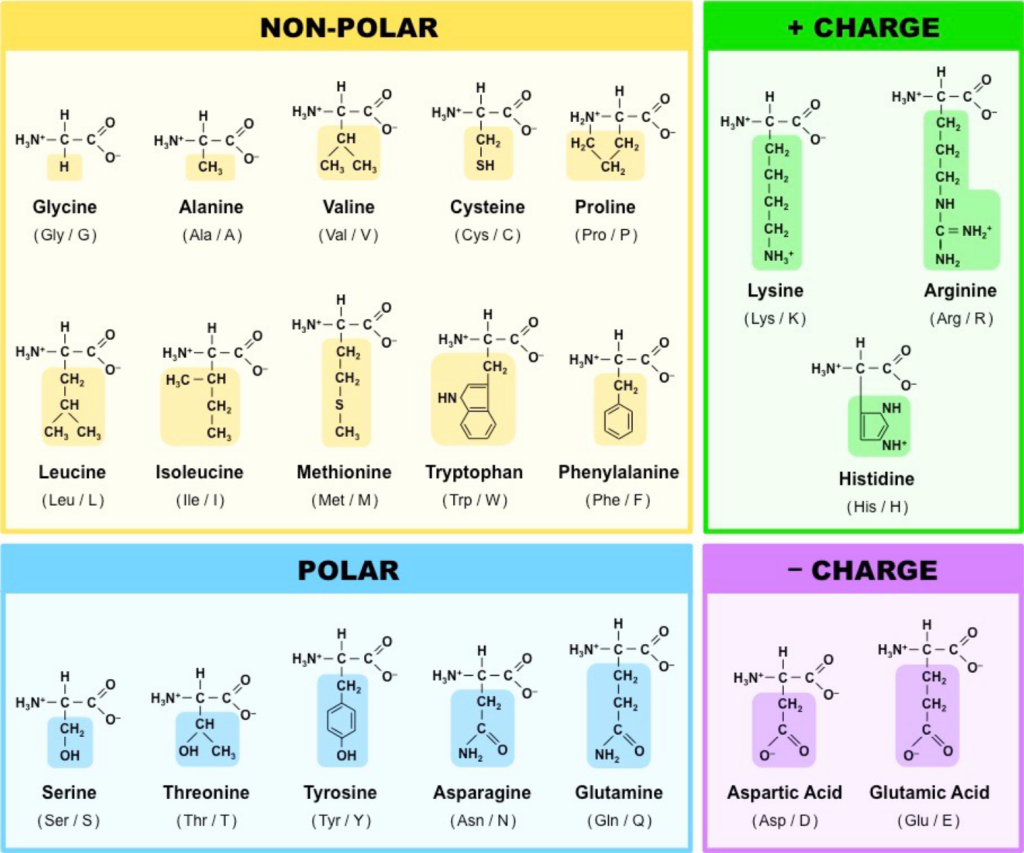
The structures of the 20 common amino acids are shown in Figure 5.8. The simplest amino acid, glycine, is shown in the upper left. The main-chain atoms of glycine are highlighted in yellow, and its R group (H) is highlighted in green. For simplicity, the main carbon is only shown in skeletal form in the image. All amino acids have the same main-chain atoms but differ in the side chains.
The R groups of amino acids contain many common groups of atoms called functional groups. Many functional groups, such as the hydroxyl group (–OH), are polar, allowing them to interact with water. This becomes important because cells are in aqueous (watery) environments. Non-polar functional groups move away from water, and groups of them can form binding pockets in a larger protein.
Learn By Doing 5.5
Amino acids can be classified by:
- the number of carbon and nitrogen bonds they contain.
- the number of carbon-carbon double bonds in their fatty acids.
- the characteristics of their side chains.
- the number of sulfur-sulfur bonds they form.
Hint: Consider the properties they contribute to the proteins they make.
Did I Get This? 5.1
Which statement does not apply to amino acids?
- They are building blocks of proteins.
- They exist primarily in charged form.
- They contain both an amino group and a carboxyl group.
- They are all polar.
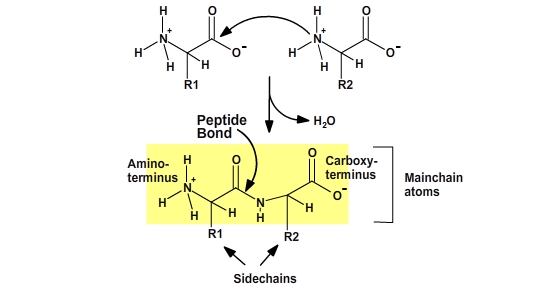
Peptide Bonds
Proteins are polymers of amino acids. The amino acids are joined together by a dehydration synthesis (sometimes called a condensation) reaction. Each amino acid in the polymer is referred to as a “residue.” Individual amino acids are joined together by the attachment of the nitrogen of an amino group of one amino acid to the carbonyl carbon (C=O) of the carboxyl group of another amino acid to create a covalent peptide bond and yield a molecule of water, as shown below.
The resulting peptide chain is linear with defined ends. Short polymers (less than 50 residues or amino acids) are usually referred to as peptides, and longer polymers as polypeptides. Several polypeptides together can form some large proteins.
Learn By Doing 5.6
Dehydration synthesis joining two amino acids results in the formation of:
- a hydrogen bond
- an ionic bond
- a dehydration bond
- a peptide bond
Hint: It is a special name given to the covalent bond between amino and carboxyl group.
Did I Get This? 5.2
The peptide bond:
- is formed between two amino groups.
- is non-polar.
- is the product of a dehydration synthesis reaction.
- is formed between two carboxyl groups.
Nucleic Acids
Learning Objectives
- Describe the structure of DNA.
- Discuss how DNA is related to protein synthesis.
Nucleic acids are primarily located in the cell nucleus (hence the name). The most important are DNA and RNA. These molecules store the cell’s “software”—genes are the instructions that govern our cells’ function, processes, and structure. Humans and other sexually reproducing species have 2 copies of almost 22,000 genes. Human DNA is organized into 46 chromosomes (23 from each parent). Genes carry the genetic code to build one’s body and are unique to each individual except identical twins.
The genetic code is comprised of ordered sequences of four nitrogen-containing bases—adenine, cytosine, guanine, and thymine (uracil in RNA), each of which is part of a nucleotide monomer. These are arranged in sets of three called triplets. Each triplet specifies an amino acid, which in turn is a component of a protein macromolecule. All the intricate complexity of the human body arises from the information encoded by just four chemicals in a single long DNA macromolecule.
In humans, mistakes in the structures of DNA and RNA cause diseases, including sickle cell anemia, hemophilia, Huntingdon’s chorea, and some types of cancer. Even a small error can result in a dramatic effect. Sickle cell disease is caused when just one amino acid in the DNA base sequence is changed.
By directing the formation of proteins, which in turn direct chemical processes, nucleic acids instruct cells on how to differentiate into various organs. All of our cells, with a few exceptions, possess the same genetic material. During development, whole sets of DNA sequences are shut down or activated to drive specific processes. These processes lead to cells with different structures and functions that form organs such as the heart, liver, skin, and brain.
Structure of RNA and DNA
As stated earlier, our genetic code is determined by only four bases in DNA (G, C, A, T), which are repeated and arranged in a particular order. For example,
1 AGCCCTCCAG GACAGGCTGC ATCAGAAGAG GCCATCAAGC AGATCACTGT CCTTCTGCCA
61 TGGCCCTGTG GATGCGCCTC CTGCCCCTGC TGGCGCTGCT GGCCCTCTGG GGACCTGACC
121 CAGCCGCAGC CTTTGTGAAC CAACACCTGT GCGGCTCACA CCTGGTGGAA GCTCTCTACC
181 TAGTGTGCGG GGAACGAGGC TTCTTCTACA CACCCAAGAC CCGCCGGGAG GCAGAGGACC
241 TGCAGGTGGG GCAGGTGGAG CTGGGCGGGG GCCCTGGTGC AGGCAGCCTG CAGCCCTTGG
301 CCCTGGAGGG GTCCCTGCAG AAGCGTGGCA TTGTGGAACA ATGCTGTACC AGCATCTGCT
361 CCCTCTACCA GCTGGAGAAC TACTGCAACT AGACGCAGCC CGCAGGCAGC CCCACACCCG
421 CCGCCTCCTG CACCGAGAGA GATGGAATAA AGCCCTTGAA CCAGCAAAA
This may seem like a random string of G, C, A, and T, but this DNA codes for human insulin. DNA is organized into a linear polymer in a double helix and maintains the inherited order of bases or genetic code. The “steps” of the DNA ladder have the code that ultimately directs the synthesis of our proteins.
The two main types of nucleic acids are deoxyribonucleic acid (DNA) and ribonucleic acid (RNA). DNA is the genetic material found in all living organisms, ranging from single-celled bacteria to multicellular mammals. This linear polymer of genetic code is maintained when double-stranded DNA is transcribed to single-stranded messenger RNA (mRNA). The DNA molecules never leave the nucleus but instead use a disposable copy made of mRNA to communicate with the rest of the cell. Messenger RNA is read by a molecular machine called the ribosome. The mRNA contains information for the order of the amino acids in a protein. Other types of RNA are also involved in protein synthesis and its regulation.
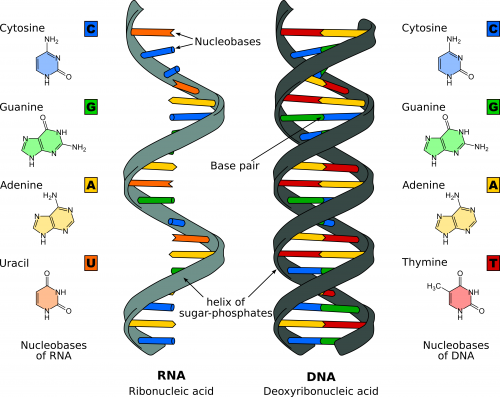
Nucleotides
DNA and RNA are made up of monomers known as nucleotides. The nucleotides combine with each other to form a polynucleotide, either DNA or RNA. Each nucleotide is made up of three components: a nitrogenous base, a pentose (five-carbon) sugar, and a phosphate group. Each nitrogenous base in a nucleotide is attached to a sugar molecule, which is attached to a phosphate group. There are four types of nucleotide found in DNA: adenine (A), thymine (T), guanine (G), and cytosine (C). In RNA, thymine is replaced by uracil (U).
Structure of a nucleotide
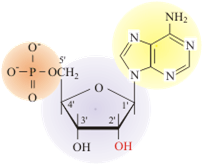
The fundamental unit of DNA is the nucleotide. The nucleotide monomer contains a phosphate group (shown in orange n Figure 5.11), eventually giving the DNA polymer its charge and interconnecting nucleotides on the backbone. The sugar group is a five-sided sugar (shown in purple in Figure 5.11). The nitrogenous base (shown in yellow n Figure 5.11) determines the type of nucleotide formed.
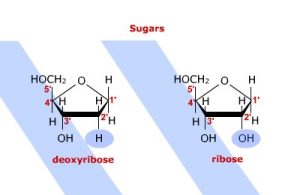
The major difference in the polymer backbones between DNA and RNA is the sugar used in the formation of the polymer. In DNA, the 2′ position of the sugar has a hydrogen atom. In RNA, the 2′ position of the sugar has an OH (hydroxyl) group and the sugar is the monosaccharide ribose.
The linkage of individual nucleotides is made by a bridging phosphate molecule between two hydroxyl groups, one on each sugar ring. The resulting polymer is a string of alternating phosphate and sugar molecules linked by covalent bonds in one very long macromolecule.
Hydrogen Bonding Between Bases
![]() |
![]() |
The alternating sugar and phosphate groups lie on the outside of each strand, forming the backbone of the DNA. The nitrogenous bases are stacked in the interior, like the steps of a staircase, and these bases pair. Thus, DNA has a spiral structure composed of two strands, or polymers, of nucleotides. The two strands are bonded to each other at their nitrogen bases with hydrogen bonds, and the strands coil about each other along their length, hence the “double helix” description, which means a double spiral.
Recall that hydrogen bonds are weak interactions, not like the covalent bonds of the phosphate-sugar backbone. Thus, DNA is held together but can be pulled apart to access the information contained in the sequence of nitrogen bases in the middle of the molecule.
To maintain an equal distance between the two strands of DNA, the larger nitrogen-containing bases must bind with the smaller nitrogen-containing bases. Specifically, A always binds with T, and G always binds with C in DNA. A useful memory device is that A and T are angular letters, and G and C are both curvy.
Copying DNA and Building Proteins
DNA replication: Every time a cell divides, all of the DNA of the genome is duplicated (a process called replication) so that each cell after the division (called a daughter cell) has the same DNA as the original cell (called the mother cell).

DNA transcription: For the genetic code to become a protein, it goes through a transcription step. DNA is transcribed into mRNA. The mRNA is then shuttled out of the nucleus to the region of protein synthesis.

RNA translation: Since nucleic acids and proteins are built from different monomers, translation between the “language” of nucleotides and the “language” of amino acids is required.

Learn By Doing 5.7
In DNA, nucleotide bonding forms a compound with a characteristic shape known as a(n) ________.
- beta chain
- pleated sheet
- alpha helix
- double helix
Compare the structure and function of DNA to the structure and function of mRNA.
Lipids
Learning Objectives
- Define the essential characteristic of a lipid.
- Describe how lipids are distributed in a cell membrane.
- Describe how amphipathic structures of lipids lead to compartmentalization.
Lipids are a diverse group of compounds united by a common feature. Lipids are hydrophobic (“water-fearing”), or insoluble in water because they are non-polar molecules. This is because they are hydrocarbons that include only non-polar carbon-carbon or carbon-hydrogen bonds.
Perhaps the most important role of lipids is in forming the membranes of cells and organelles. In this way, lipids enable the isolation and control of chemical processes. They also play a role in energy storage and cell signaling.
Cells store energy for long-term use in the form of lipids called fats. Lipids also provide insulation from the environment for plants and animals. For example, they help keep aquatic birds and mammals dry because of their water-repelling nature. Lipids are also the building blocks of many hormones and are an important constituent of the plasma membrane. Lipids include fats, oils, waxes, phospholipids, and steroids.
A fat molecule, such as a triglyceride, consists of two main components—glycerol and fatty acids. Glycerol is an organic compound with three carbon atoms, five hydrogen atoms, and three hydroxyl (–OH) groups. Fatty acids have a long chain of hydrocarbons to which an acidic carboxyl group is attached, hence the name “fatty acid.” The number of carbons in the fatty acid may range from 4 to 36; the most common are those containing 12–18 carbons. In a fat molecule, a fatty acid is attached to each of the three oxygen atoms in the –OH groups of the glycerol molecule with covalent bonds, which are made up of fatty acids and glycerol, phospholipids, and steroids.
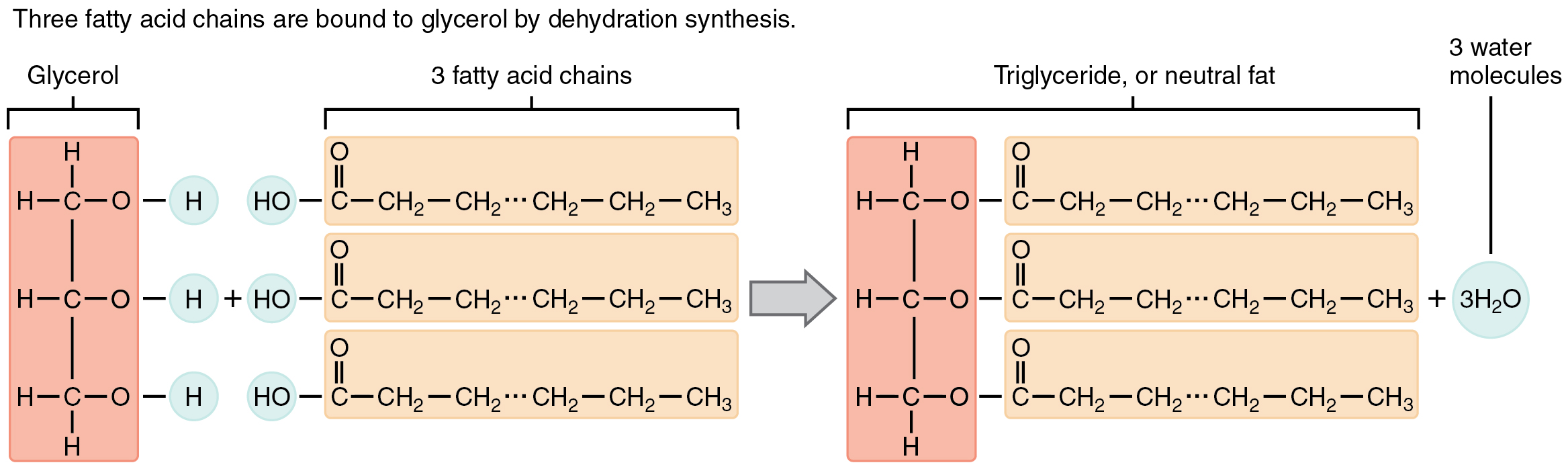
During this covalent bond formation, three water molecules are released. The three fatty acids in the fat may be similar or dissimilar. These fats are also called triglycerides because they have three fatty acids. Some fatty acids have common names that specify their origin. For example, palmitic acid, a saturated fatty acid, is derived from the palm tree. Arachidic acid is derived from Arachis hypogaea, the scientific name for peanuts.
Fatty acids may be saturated or unsaturated. In a fatty acid chain, if there are only single bonds between neighboring carbons in the hydrocarbon chain, the fatty acid is saturated. Saturated fatty acids are saturated with hydrogen; in other words, the number of hydrogen atoms attached to the carbon skeleton is maximized. The fatty acid is unsaturated when the hydrocarbon chain contains a double bond.
Most unsaturated fats are liquid at room temperature and are called oils. If there is one double bond in the molecule, then it is known as a monounsaturated fat (e.g., olive oil), and if there is more than one double bond, then it is known as a polyunsaturated fat (e.g., canola oil).
Saturated fats tend to get packed tightly and are solid at room temperature. Animal fats with stearic acid and palmitic acid contained in meat, and fat with butyric acid contained in butter, are examples of saturated fats. Mammals store fats in specialized cells called adipocytes, where globules of fat occupy most of the cell. In plants, fat or oil is stored in seeds and is used as a source of energy during embryonic development.
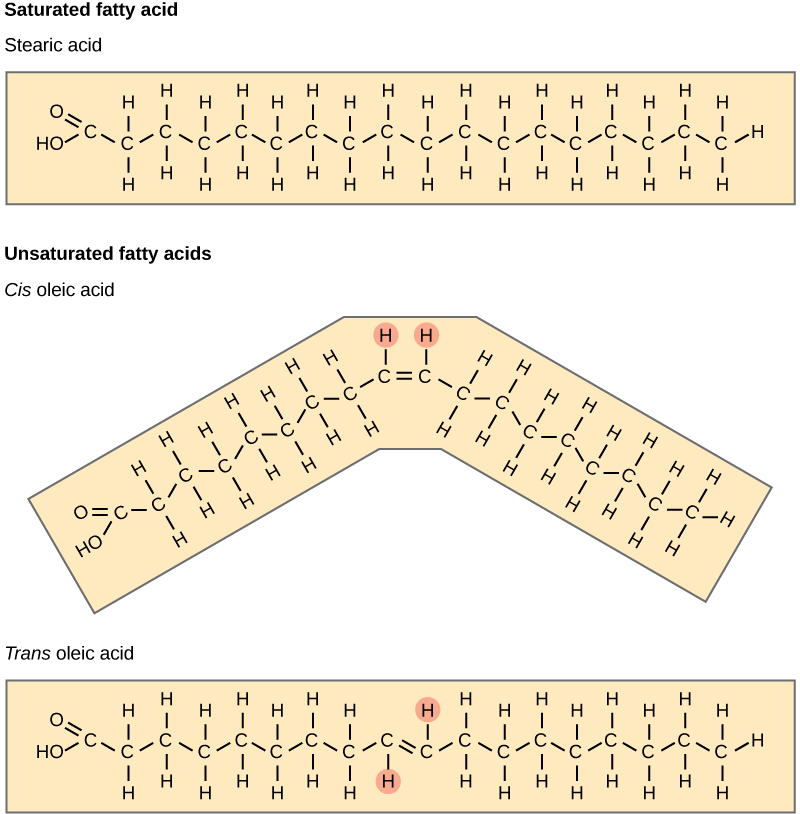
Unsaturated fats or oils are usually of plant origin and contain unsaturated fatty acids. The double bond causes a bend or a “kink” that prevents the fatty acids from packing tightly, keeping them liquid at room temperature. Olive oil, corn oil, canola oil, and cod liver oil are examples of unsaturated fats. Unsaturated fats help to improve blood cholesterol levels, whereas saturated fats contribute to plaque formation in the arteries, which increases the risk of a heart attack.
In the food industry, oils are artificially hydrogenated to make them semi-solid, leading to less spoilage and increased shelf life. Simply speaking, hydrogen gas is bubbled through oils to solidify them. During this hydrogenation process, double bonds of the conformation in the hydrocarbon chain may be converted to double bonds in the trans-conformation. This forms a trans-fat from a cis-fat. The orientation of the double bonds affects the chemical properties of the fat. During the hydrogenation process, the orientation around the double bonds is changed, making a trans-fat from a cis-fat. This changes the chemical properties of the molecule.
Margarine, some types of peanut butter, and shortening are examples of artificially hydrogenated trans-fats. Recent studies have shown that an increase in trans-fats in the human diet may lead to an increase in levels of low-density lipoprotein (LDL), or “bad” cholesterol, which, in turn, may lead to plaque deposition in the arteries, resulting in heart disease. Many fast food restaurants have recently eliminated the use of trans-fats, and U.S. food labels are now required to list their trans-fat content.
Essential fatty acids are fatty acids that are required but not synthesized by the human body. Consequently, they must be supplemented through the diet. Omega-3 fatty acids fall into this category and are one of only two known essential fatty acids for humans (the other being omega-6 fatty acids). They are a type of polyunsaturated fat and are called omega-3 fatty acids because the third carbon from the end of the fatty acid participates in a double bond.
Salmon, trout, and tuna are good sources of omega-3 fatty acids. Omega-3 fatty acids are important in brain function and normal growth and development. They may also prevent heart disease and reduce the risk of cancer.
Like carbohydrates, fats have received a lot of bad publicity. It is true that eating an excess of fried foods and other “fatty” foods leads to weight gain. However, fats do have important functions. Fats serve as long-term energy storage. They also provide insulation for the body. Therefore, “healthy” unsaturated fats in moderate amounts should be consumed on a regular basis.
Like carbohydrates, lipids are vital energy storage molecules. Carbohydrates can be used right away, and lipids provide long-term energy storage. Lipids accumulate in adipose cells (fat cells) in the body. As part of the catabolic process, from the days when humans had to forage for food, excess carbohydrates can be converted into lipids, which are then stored in fatty tissue. Ultimately, too many ingested carbohydrates and lipids lead to obesity.
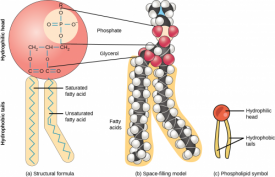
(Credit: Open Stax, CC BY)
Phospholipids
Phospholipids are the major constituent of the plasma membrane. Like fats, they are composed of fatty acid chains attached to a glycerol or similar backbone. However, instead of three fatty acids attached to the glycerol molecule, there are two fatty acids, and the glycerol backbone’s third carbon is bound to a phosphate group. Phospholipids have a hydrophilic “head” and hydrophobic “tail” (“hydro” means water, and “philo” means to love; “hydro” means water, “phobic” means fear). The fatty acid chains are hydrophobic and exclude themselves from water, whereas the phosphate is hydrophilic and interacts with water. A phospholipid bilayer is formed when the two layers of phospholipid molecules organize with the hydrophobic tails meeting in the middle.
Scientists believe that the formation of cell-like globules of lipids was a vital precursor to the origin of cellular life since membranes physically separate intracellular components from the extracellular environment. Thus, lipid membranes enclose other macromolecules, confine volumes to increase the possibility of reactions, and protect chemical processes. Proteins with hydrophobic regions float within the lipid bilayer. These molecules govern the transport of charged or lipophobic molecules in and out of the cell, such as energy molecules and waste products. Some of these lipids also have attached carbohydrate molecules jutting out of the membrane are important for cell recognition, as mentioned previously.

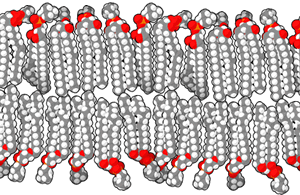
Bensaccount, Public Domain)
Phospholipids are referred to as amphipathic molecules. Such molecules have a distinct non-polar or hydrophobic region and a distinct polar region. They do not form true solutions in water. Rather, the non-polar parts are forced together, leaving the polar part of the molecule to interact with water. Both detergents and phospholipids are examples of amphipathic molecules, and phospholipids are the most common molecules in cell membranes.
Steroids and Waxes
Unlike the phospholipids and fats discussed earlier, steroids have a ring structure. Although they do not resemble other lipids, they are grouped with them because they are also hydrophobic. All steroids have four, linked carbon rings, and several of them, like cholesterol, have a short tail.
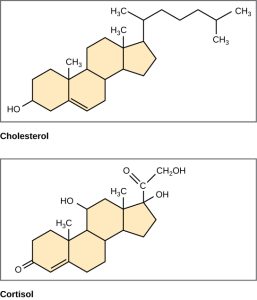
Cholesterol is a steroid. Cholesterol is mainly synthesized in the liver and is the precursor of many steroid hormones, such as testosterone and estradiol. It is also the precursor of vitamins E and K. Cholesterol is the precursor of bile salts, which help in the breakdown of fats and their subsequent absorption by cells. Although cholesterol is often spoken of in negative terms, it is necessary for the proper functioning of the body. It is a key component of the plasma membranes of animal cells.
Waxes are made up of a hydrocarbon chain with an alcohol (–OH) group and a fatty acid. Examples of animal waxes include beeswax and lanolin. Plants also have waxes, such as the coating on their leaves, that helps prevent them from drying out.
Learn By Doing 5.8
Section Summary
Living things are carbon-based. The four covalent bonding positions of the carbon atom can give rise to a wide diversity of compounds with many functions, accounting for the importance of carbon in living things.
Carbohydrates are a group of macromolecules that are a vital energy source for the cell, provide structural support to many organisms, and can be found on the surface of the cell as receptors or for cell recognition. Carbohydrates are classified as monosaccharides, disaccharides, and polysaccharides, depending on the number of monomers in the molecule.
Proteins are a class of macromolecules that can perform a diverse range of functions for the cell. They help metabolism by providing structural support and acting as enzymes, carriers, or hormones. The building blocks of proteins are amino acids. Proteins are organized at four levels: primary, secondary, tertiary, and quaternary. Protein shape and function are intricately linked; any change in shape caused by changes in temperature, pH, or chemical exposure may lead to protein denaturation and a loss of function.
Nucleic acids are molecules made up of repeating units of nucleotides that direct cellular activities such as cell division and protein synthesis. Each nucleotide is made up of a five-carbon sugar, a nitrogenous base, and a phosphate group. There are two types of nucleic acids: DNA and RNA.
Lipids are a class of macromolecules that are non-polar and hydrophobic in nature. Major types include fats and oils, waxes, phospholipids, and steroids. Fats and oils are a stored form of energy and can include triglycerides. Fats and oils are usually made up of fatty acids and glycerol.
Virtually every task performed by living organisms requires energy. Energy is needed to perform heavy labor and exercise, but humans also use energy while thinking and even during sleep. In fact, the living cells of every organism constantly use energy. Nutrients and other molecules are imported into the cell, metabolized (broken down), and possibly synthesized into new molecules, modified if needed, transported around the cell, and possibly distributed to the entire organism. For example, the large proteins that make up muscles are built from smaller molecules imported from dietary amino acids. Complex carbohydrates are broken down into simple sugars that the cell uses for energy. Just as energy is required to both build and demolish a building, energy is required for the synthesis and breakdown of molecules as well as the transport of molecules into and out of cells. In addition, processes such as ingesting and breaking down pathogenic bacteria and viruses, exporting wastes and toxins, and movement of the cell require energy. From where and in what form does this energy come? How do living cells obtain energy, and how do they use it? This chapter will discuss different forms of energy and the physical laws that govern energy transfer. This chapter will also describe how cells use energy and replenish it and how chemical reactions in the cell are performed with great efficiency.
Energy and Metabolism
Learning Objectives
- Define metabolism.
- Define energy.
- Describe how the laws governing energy apply to human biology.
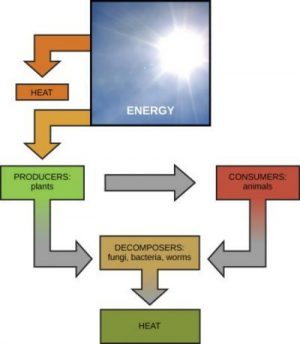
Scientists use the term bioenergetics to describe the concept of energy flow through living systems, such as cells. Cellular processes, such as the building and breaking down of complex molecules, occur through stepwise chemical reactions. Some of these chemical reactions are spontaneous and release energy, whereas others require energy to proceed. Just as living things must continually consume food to replenish their energy supplies, cells must continually produce more energy to replenish that used by the many energy-requiring chemical reactions that constantly take place. Together, all of the chemical reactions that take place inside cells, including those that consume or generate energy, are referred to as the cell’s metabolism.
Energy
Learning Objectives
- Define energy.
- Describe how the laws governing energy apply to human biology.
Scientists have studied energy and energy transfer involving physical matter in a branch of science known as thermodynamics. The matter being considered in a particular case of energy transfer is called a system, and everything outside of that matter is called the surroundings. For instance, when heating a pot of water on the stove, the system includes the stove, the pot, and the water. Energy is transferred within the system (between the stove, pot, and water). There are two types of systems: open and closed. In an open system, energy can be exchanged with its surroundings. The stovetop system is open because heat can be lost to the air. A closed system cannot exchange energy with its surroundings.
Biological organisms are open systems. Energy is exchanged between them and their surroundings as they use energy from the sun to perform photosynthesis or consume energy-storing molecules and release energy to the environment by doing work and releasing heat. Like all things in the physical world, energy is subject to physical laws. The laws of thermodynamics govern the transfer of energy in and among all systems in the universe.
In general, energy is defined as the ability to do work or create change. Energy exists in different forms. For example, electrical energy, light energy, and heat energy are all different types of energy. It is important to understand two physical laws governing energy to appreciate how energy flows into and out of biological systems, including the system that is the human body.
The first law of thermodynamics states that the total amount of energy in the universe is constant and conserved. In other words, there has always been, and always will be, exactly the same amount of energy in the universe. Energy exists in many different forms. According to the first law of thermodynamics, energy may be transferred from place to place or transformed into different forms, but it cannot be created or destroyed. The transfers and transformations of energy take place around us all the time. Light bulbs transform electrical energy into light and heat energy. Gas stoves transform chemical energy from natural gas into heat energy. Plants perform one of the most biologically useful energy transformations on earth: converting the energy of sunlight to chemical energy stored within organic molecules. Some examples of energy transformations are shown below.
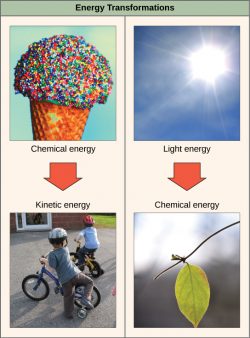
The challenge for all living organisms is to obtain energy from their surroundings in forms that they can transfer or transform into usable energy to do work. Living cells have evolved to meet this challenge. Chemical energy stored within organic molecules such as sugars and fats is transferred and transformed through a series of cellular chemical reactions into energy within molecules of ATP. (We’ll discuss ATP shortly). Energy in ATP molecules is easily accessible to do work. Examples of the types of work that cells need to do include building complex molecules, transporting materials, powering the motion of cilia or flagella, and contracting muscle fibers to create movement.
A living cell’s primary tasks of obtaining, transforming, and using energy to do work may seem simple. However, the second law of thermodynamics explains why these tasks are harder than they appear. The second law of thermodynamics says that energy transfers or transformations, energy will always be lost from the system as heat. Another way of saying this is that no energy transfer or transformation is ever completely efficient. In every energy transfer, some amount of energy is lost in a form that is unusable. In most cases, this form is heat energy. Thermodynamically, heat energy is defined as the energy transferred from one system to another that does not work. For example, when a light bulb is turned on, some of the energy being converted from electrical energy into light energy is lost as heat energy. Likewise, some energy is lost as heat energy during cellular metabolic reactions.
Learn By Doing 5.9
Define energy.
How is the definition provided in the text different from, and similar to, how you have thought about energy?
Which of the following, if any, is not an example of an energy transformation?
- Heating up dinner in a microwave
- Solar panels at work
- Formation of static electricity
- None of the above
Apply the second law of thermodynamics to a living organism such as a human being.
Hint: What would be the consequences if that person became a closed system?
Potential and Kinetic Energy
Learning Objectives
- Explain the difference between kinetic and potential energy.
- Distinguish between activation energy and free energy.
- Describe the differences between endergonic and exergonic reactions.
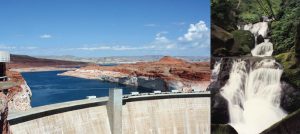
When an object is in motion, there is energy associated with that object. Think of a wrecking ball. Even a slow-moving wrecking ball can do a great deal of damage to other objects. Energy associated with objects in motion is called kinetic energy. A speeding bullet, a walking person, and the rapid movement of molecules in the air (which produces heat) all have kinetic energy.
Now, what if that same motionless wrecking ball is lifted two stories above ground with a crane? If the suspended wrecking ball is unmoving, is there energy associated with it? The answer is yes. The energy required to lift the wrecking ball did not disappear but is now stored in the wrecking ball by its position and the force of gravity acting on it. This type of energy is called potential energy. If the ball were to fall, the potential energy would be transformed into kinetic energy until all of the potential energy was exhausted when the ball rested on the ground. Wrecking balls also swing like a pendulum; through the swing, there is a constant change of potential energy (highest at the top of the swing) to kinetic energy (highest at the bottom of the swing). Other examples of potential energy include the energy of water held behind a dam or a person about to skydive out of an airplane.
Potential energy is not only associated with the location of matter but also with the structure of matter. Even a spring on the ground has potential energy if it is compressed; so does a rubber band that is pulled taut. On a molecular level, the bonds that hold the atoms of molecules together exist in a particular structure that has potential energy. Remember that anabolic cellular pathways require energy to synthesize complex molecules from simpler ones, and catabolic pathways release energy when complex molecules are broken down. The fact that energy can be released by the breakdown of certain chemical bonds implies that those bonds have potential energy. In fact, there is potential energy stored within the bonds of all the food molecules we eat, which is eventually harnessed for use. This is because these bonds can “release” energy when broken. The type of potential energy that exists within chemical bonds, and is released when those bonds are broken, is called chemical energy. Chemical energy is responsible for providing living cells with energy from food. The release of energy occurs when the molecular bonds within food molecules are broken.
Free Energy
After learning that chemical reactions release energy when energy-storing bonds are broken, an important next question is: How can the energy released from one reaction be compared to that of another? A measurement of free energy is used to quantify these energy transfers. Recall that according to the second law of thermodynamics, all energy transfers involve the loss of some amount of energy in an unusable form, such as heat. Free energy specifically refers to the energy associated with a chemical reaction that is available after the losses are accounted for. In other words, free energy is usable energy or energy that is available to do work.
If energy is released during a chemical reaction, then the change in free energy, signified as ∆G (delta G) will be a negative number. A negative change in free energy also means that the products of the reaction have less free energy than the reactants because they release some free energy during the reaction. Reactions that have a negative change in free energy and consequently release free energy are called exergonic reactions. Think: exergonic means energy is exiting the system. These reactions are also referred to as spontaneous reactions, and their products have less stored energy than the reactants. An important distinction must be drawn between the term spontaneous and the idea of a chemical reaction occurring immediately. Contrary to the everyday use of the term, a spontaneous reaction is not one that suddenly or quickly occurs. The rusting of iron is an example of a spontaneous reaction that occurs slowly, little by little, over time.
If a chemical reaction absorbs energy rather than releases energy on balance, then the ∆G for that reaction will be a positive value. In this case, the products have more free energy than the reactants. Thus, these reactions’ products can be considered energy-storing molecules. These chemical reactions are called endergonic reactions, and they are non-spontaneous. An endergonic reaction will not take place on its own without the addition of free energy.
Activation Energy
Another important concept must be considered regarding endergonic and exergonic reactions. Exergonic reactions require a small amount of energy input to get going before they can proceed with their energy-releasing steps. These reactions have a net release of energy but still require some energy input in the beginning. This small amount of energy input necessary for all chemical reactions to occur is called activation energy.
Learn By Doing 5.10
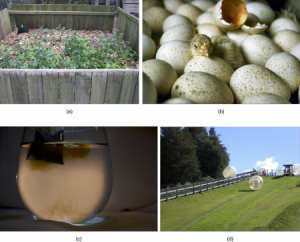
The images to the right are examples of endergonic processes (ones that require energy) and exergonic processes (ones that release energy).
Image (a) is a compost pile, image (b) is a baby chick emerging from a fertilized egg, image (c) shows a teabag’s dark-colored contents diffusing into a clear mug of water, and image (d) shows a large ball rolling downhill.(credit a: modification of work by Natalie Maynor; credit b: modification of work by USDA; credit c: modification of work by Cory Zanker; credit d: modification of work by Harry Malsch)
Which pictures represent endergonic and which exergonic processes? Why?
Enzymes
Learning Objective
- Define the terms active site and substrate.
- Describe how enzymes function as molecular catalysts by lowering the activation energy.
A substance that helps a chemical reaction to occur is called a catalyst, and the molecules that catalyze biochemical reactions are called enzymes. Most enzymes are proteins and perform the critical task of lowering the activation energies of chemical reactions inside the cell.
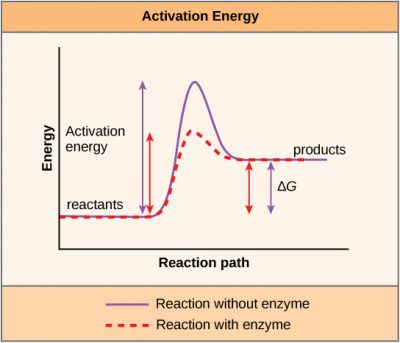
Most of the reactions critical to a living cell happen too slowly at normal temperatures to be of any use to the cell. Without enzymes to speed up these reactions, life could not persist. Enzymes do this by binding to the reactant molecules and holding them in such a way as to make the chemical bond-breaking and -forming processes take place more easily. It is important to remember that enzymes do not change whether a reaction is exergonic (spontaneous) or endergonic. This is because they do not change the free energy of the reactants or products. They only reduce the activation energy required for the reaction to go forward. In addition, an enzyme itself is unchanged by the reaction it catalyzes; it is not “used up.” Once one reaction has been catalyzed, the enzyme is able to participate in other reactions.
The chemical reactants to which an enzyme binds are called the enzyme’s substrates. Depending on the particular chemical reaction, there may be one or more substrates. A single reactant substrate is broken down into multiple products in some reactions. In others, two substrates may come together to create one larger molecule. Two reactants might also enter a reaction and become modified, leaving the reaction as two products. The enzyme’s active site is located within the enzyme where the substrate binds. The active site is where the “action” happens. Since enzymes are proteins, there is a unique combination of amino acid side chains within the active site. Each side chain is characterized by different properties. They can be large or small, weakly acidic or basic, hydrophilic or hydrophobic, positively or negatively charged, or neutral. The unique combination of side chains creates a very specific chemical environment within the active site. This specific environment is suited to bind to one specific chemical substrate (or substrates). Thus, each reaction is helped along by a different enzyme.
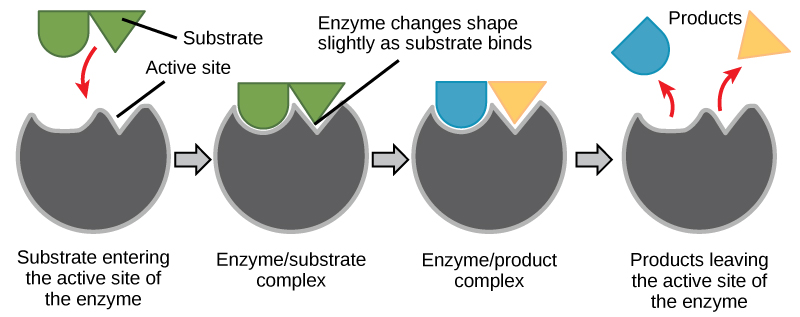
Active sites are subject to the influences of the local environment. Increasing the environmental temperature generally increases reaction rates, enzyme-catalyzed or otherwise. However, temperatures outside of an optimal range reduce the rate at which an enzyme catalyzes a reaction. Hot temperatures will eventually cause enzymes to denature, an irreversible change in the three-dimensional shape and, therefore the function of the enzyme. Enzymes are also suited to function best within a certain pH, and salt concentration range, and, as with temperature, extreme pH and salt concentrations can cause enzymes to denature.
Enzymes can also be regulated in ways that either promote or reduce enzyme activity. There are many kinds of molecules that inhibit or promote enzyme function and various mechanisms by which they do so. In some cases of enzyme inhibition, an inhibitor molecule is similar enough to a substrate that it can bind to the active site and simply block the substrate from binding. When this happens, the enzyme is inhibited through competitive inhibition because an inhibitor molecule competes with the substrate to bind to the active site.
Learn By Doing 5.11
Which of the following is not true about enzymes?
- They are consumed (used up) by the reactions they catalyze.
- They are usually made of amino acids.
- They lower the activation energy of chemical reactions.
- Each one is specific to the particular substrate(s) to which it binds.
Why are enzymes so important to the function of cells?
Learning Objectives
- Explain what metabolic pathways are.
- Apply the terms anabolic and catabolic to a metabolism.
Metabolic Pathways
Consider the metabolism of sugar. This is a classic example of one of the many cellular processes that use and produce energy. Living things consume sugars as a major energy source because sugar molecules have a great deal of energy stored within their bonds. For the most part, photosynthesizing organisms like plants produce these sugars. During photosynthesis, plants use energy (originally from sunlight) to convert carbon dioxide gas (CO2) into sugar molecules (like glucose: C6H12O6). They consume carbon dioxide and produce oxygen as a waste product. This reaction is summarized as:
6 CO2 + 6 H2O
Because this process involves synthesizing an energy-storing molecule, it requires energy input to proceed. During the light reactions of photosynthesis, energy is provided by a molecule called adenosine triphosphate (ATP), which is the primary energy currency of all cells. Just as the dollar is used as currency to buy goods, cells use molecules of ATP as energy currency to perform immediate work. In contrast, energy-storage molecules such as glucose are consumed only to be broken down to use their energy. The reverse reaction to photosynthesis summarizes the reaction that harvests the energy of a sugar molecule in cells requiring oxygen to survive. In this reaction, oxygen is consumed, and carbon dioxide is released as a waste product. The reaction is summarized as:
C6H12O6 + 6 O2 −−> 6 H2O + 6 CO2
Both of these reactions involve many steps, and the reaction above is a summary equation showing the results of those steps. We will explore this topic further in the next chapter.
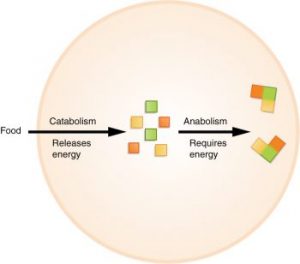
The processes of making and breaking down sugar molecules are two examples of metabolic pathways. A metabolic pathway is a series of chemical reactions that takes a starting molecule and modifies it, step by step, through a series of metabolic intermediates, eventually yielding a final product. In the example of sugar metabolism, the first metabolic pathway synthesized sugar from smaller molecules, and the other pathway broke sugar down into smaller molecules. These two opposite processes—the first requiring energy and the second producing energy—are referred to as anabolic pathways (building polymers) and catabolic pathways (breaking down polymers into their monomers), respectively. Consequently, metabolism is composed of synthesis (anabolism) and degradation (catabolism).
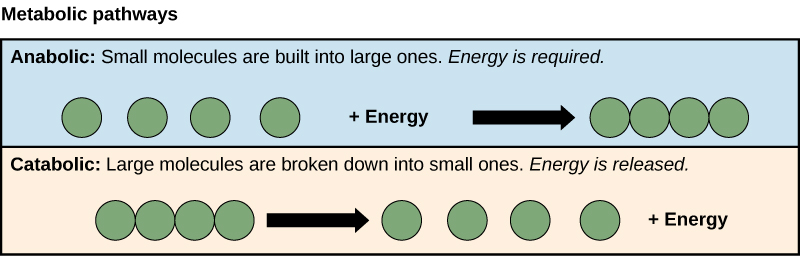
It is important to know that the chemical reactions of metabolic pathways do not take place on their own. Each reaction step is facilitated, or catalyzed, by a protein called an enzyme. Enzymes are important for catalyzing all types of biological reactions—those that require energy and release energy.
Learn By Doing 5.12
Does physical exercise that increases muscle mass involve anabolic and/or catabolic processes? Give evidence for your answer.
Hint: Consider what you observe when someone is lifting weights, a common muscle-building exercise.
Section Summary
Cells perform the functions of life through various chemical reactions. A cell’s metabolism refers to the combination of chemical reactions that take place within it. Catabolic reactions break down complex chemicals into simpler ones and are associated with energy release. Anabolic processes build complex molecules out of simpler ones and require energy.
In studying energy, the term system refers to the matter and environment involved in energy transfers. Entropy is a measure of the disorder of a system. The physical laws that describe the transfer of energy are the laws of thermodynamics. The first law states that the total amount of energy in the universe is constant. The second law of thermodynamics states that every energy transfer involves some loss of energy in an unusable form, such as heat energy. Energy comes in different forms: kinetic, potential, and free. The change in free energy of a reaction can be negative (releases energy, exergonic) or positive (consumes energy, endergonic). All reactions require an initial input of energy to proceed, called activation energy.
Enzymes are chemical catalysts that speed up chemical reactions by lowering their activation energy. Enzymes have an active site with a unique chemical environment that fits particular chemical reactants for that enzyme, called substrates. Enzyme action is regulated to conserve resources and respond optimally to the environment.
*
“Learn By Doing” and “Did I Get This?” Feedback
Learn By Doing 5.1
Identify the 4 major classes of macromolecules.
Nucleic acids, proteins, carbohydrates, and lipids are the 4 major classes of macromolecules.
Compare and contrast organic and inorganic molecules.
Both organic and inorganic molecules may contain carbon, hydrogen, and/or other elements. However, organic molecules are defined as any molecules that contain 2 or more hydrogen atoms covalently bound to carbon.
Dehydration synthesis leads to the formation of
- monomers
- polymers
- water and polymers
- none of the above
During the breakdown of polymers, which of the following reactions takes place?
- hydrolysis
- dehydration
- condensation
- covalent bond
Describe the differences between dehydration synthesis and hydrolysis.
Dehydration synthesis is an anabolic (build-up) reaction that produces more complex molecules from simpler ones. Water and polymers are reactants, while a polymer and water are products.
Hydrolysis is a catabolic (break-down) reaction that produces simpler molecules from more complex ones. In the context of macromolecules, monomers are the reactants, while water and a polymer are the products.
Identify the 4 broad classes of enzymes used to speed up dehydration synthesis and hydrolysis reactions.
- Nucleases facilitate nucleic acid breakdown (hydrolysis) or build-up (dehydration synthesis).
- Proteases facilitate the breakdown (hydrolysis) or build-up (dehydration synthesis) of proteins.
- Carbohydrases facilitate carbohydrates’ breakdown (hydrolysis) or build-up (dehydration synthesis).
- Lipases facilitate lipids’ breakdown (hydrolysis) or build-up (dehydration synthesis).
Learn By Doing 5.2
Each carbon molecule can bond with as many as ________ other atom(s) or molecule(s).
- one
- two
- six
- four
Describe why carbon is well-suited to form the “backbone” of organic molecules.
Carbon is unique and found in all living things because it can form up to four covalent bonds between atoms or molecules. These can be nonpolar or polar covalent bonds, and they allow for the formation of long chains of carbon molecules that combine to form proteins and DNA.
Why are biological macromolecules considered organic?
Biological macromolecules are organic because they contain multiple carbon-hydrogen covalent bonds.
Indicate whether the following chemical formulas represent organic or inorganic compounds.
- C6H12O6 (Glucose)
- H2O (Water)
- KI (Potassium iodide)
- MgSO4 (Magnesium sulfate)
- CH4O (Methanol)
Judging from these formulas, both methanol and glucose are organic molecules since it seems likely that there are at least 2 covalent bonds between carbon and hydrogen atoms.
Examine the images below. Which molecules would you classify as organic? Explain your answers in terms of the definition of an organic molecule.
Hint: Remember that you can determine the molecular formula from the diagram, though you may not need to.
Learn By Doing 5.3
Cellulose, glycogen, and starch are examples of ________.
- monosaccharides
- disaccharides
- lipids
- polysaccharides
Cellulose, glycogen, and starch are all constructed from the same monomer. Describe how they can have different functions, given that they are all made of the same subunit.
Cellulose, glycogen, and starch are all constructed from glucose monomers. their different functions arise because of the way the glucose molecules are strung together into a polymer. Cellulose functions as a structural molecule in plants. This is possible because every other glucose molecule is “upside down.” This allows for hydrogen bonding between adjacent cellulose chains. In addition, cellulose is a linear molecule without the branching observed in glycogen and starch. Glycogen in animals and starch in plants function as short-term energy storage. The glucose monomers are linked together with the carbon rings facing the same direction in both starch and glycogen. This means it is easier to break off glucose monomers when glucose is required for cellular respiration.
Learn By Doing 5.4
If a protein has a quaternary structure, that means it:
- Is a small protein
No: In fact, more proteins with quaternary structures are large, although this is not a necessity. - Is made of all 20 amino acids
No: Although it is likely that a protein will contain most or all of the amino acids, it is not a necessity. - Is most likely coded for by multiple genes
Yes: Quaternary structure indicates multiple amino acid chains combining into a single, larger protein. Each amino acid chain is typically coded by a separate gene. For example, hemoglobin is made of alpha chains and beta chains, which are coded by separate genes. - Has enzymatic activity
No: Many proteins with quaternary structure are not enzymes, but function as transport proteins, etc.
Different cell types express different types and concentrations of proteins. You would expect a cell common to connective tissues like ligaments and tendons to produce an abundance of proteins with which of the following functions?
- Structural
Yes: Tendons and ligaments are structural connective tissues. The mast cells of these tissues produce and secrete many structural proteins that maintain the strength of these tissues. - Cell signaling
No: Although all cells produce some proteins that are involved in cell signaling, you would not expect a connective tissue cell in ligaments and tendons to produce more of these than the typical cell. - Enzymatic
No: Although all cells produce enzymatic proteins, you would not expect a connective tissue cell in ligaments and tendons to produce more of these than the typical cell. - Immune
No: Some connective tissue does contain an abundance of immune cells that produce immune proteins, but that would not be the case for tendons or ligaments.
Learn By Doing 5.5
Amino acids can be classified by:
- the number of carbon and nitrogen bonds they contain.
No: Most contain 1 carbon-nitrogen bond. - the number of carbon-carbon double bonds in their fatty acids.
No: Amino acids do not contain fatty acids. - the characteristics of their side chains.
Yes: Side chains can be classified as polar (charged or uncharged), nonpolar, etc. - the number of sulfur-sulfur bonds they form.
No: Sulfur-sulfur bonds occur between certain amino acids, not within them.
Did I Get This? 5.1
Which statement does not apply to amino acids?
- They are building blocks of proteins.
No: This statement is correct. Amino acids are the protein monomers, making them the building blocks. - They exist primarily in charged form.
No: This statement is correct. Both the amino and carboxyl group can commonly be found in charged form. - They contain both an amino group and a carboxyl group.
No:This statement is correct. Each amino acid does contain an amino and carboxylic acid group, which is where they derive their name from. - They are all polar.
Yes: This statement is not true. Not all amino acids are polar, several are non-polar.
Learn By Doing 5.6
Dehydration synthesis joining two amino acids results in the formation of:
- a hydrogen bond
No: The bond between amino acids after a dehydration synthesis is a form of covalent bond, not a hydrogen bond. - an ionic bond
No: Dehydration synthesis results in a covalent bond, not an ionic bond. - a dehydration bond
No: Dehydration can be used to describe a form of synthesis, but not a resulting bond. - a peptide bond
Yes: Peptide bonds form between the amino and carboxyl groups of different amino acids.
Did I Get This? 5.2
The peptide bond:
- is formed between two amino groups.
No: If amino acids combined between amino groups, that wouldn’t leave any amino groups left to elongate the chain of amino acids. - is non-polar.
No: The peptide bond occurs between the more electronegative nitrogen and less electronegative carbon, making it a polar bond. - is the product of a dehydration synthesis reaction.
Yes: By using one amino and one carboxyl group from each amino acid, the chain can continue to elongate. - is formed between two carboxyl groups.
No: If amino acids combined between carboxyl groups, it wouldn’t leave any carboxyl groups left to elongate the chain of amino acids.
Learn By Doing 5.7
In DNA, nucleotide bonding forms a compound with a characteristic shape known as a(n) ________.
- beta chain
- pleated sheet
- alpha helix
- double helix
Compare the structure and function of DNA to the structure and function of mRNA.
Our Answer: DNA has a double-helix structure. The sugar and the phosphate are on the outside of the helix and the nitrogenous bases are in the interior. The 2 strands are held together by hydrogen bonds between the nitrogenous bases. The monomers of DNA are nucleotides containing deoxyribose, one of the four nitrogenous bases (A, T, G, and C), and a phosphate group. DNA codes for messenger RNA (mRNA). RNA is usually single-stranded and is made of ribonucleotides. A ribonucleotide contains ribose (the pentose sugar), one of the four nitrogenous bases (A, U, G, and C), and the phosphate group. mRNA codes for amino acids, the monomers of proteins.
Learn By Doing 5.8
Fat serves as a valuable way for animals to store energy. It can also provide insulation. Waxes can protect fur from getting wet. Phospholipids and steroids are important parts of animal cell membranes. Some steroids also act as hormones, long-distance chemical messengers.
Learn By Doing 5.9
Define energy.
How is the definition provided in the text different from, and similar to, how you have thought about energy?
Energy is what is required to do work. (Work is defined as anything that changes the form or position of an object.)
Which of the following, if any, is not an example of an energy transformation?
- Heating up dinner in a microwave
- Solar panels at work
- Formation of static electricity
- None of the above
Apply the second law of thermodynamics to a living organism such as a human being.
The second law of thermodynamics applies to living organisms such as humans because it explains why we must continually take in food to survive. Food provides high-quality chemical energy and allows us to remain open systems.
Learn By Doing 5.10
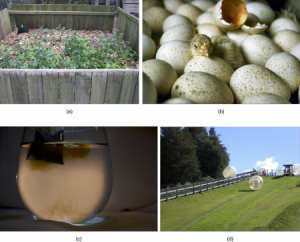
The images below are examples of endergonic processes (ones that require energy) and exergonic processes (ones that release energy). Which pictures represent endergonic and which exergonic processes? Why?
(credit a: modification of work by Natalie Maynor; credit b: modification of work by USDA; credit c: modification of work by Cory Zanker; credit d: modification of work by Harry Malsch)
Our Answer: The compost pile, tea bag, and rolling balls are examples of exergonic processes (ones that release energy). This is because in all three cases the system has less potential energy at the end of the process than in the beginning. The baby chick and plants growing from the compost pile are examples of endergonic processes (ones that require energy). This is because in living systems (especially during growth) the system has more potential energy at the end of the process than in the beginning.
Learn By Doing 5.11
Which of the following is not true about enzymes?
- They are consumed (used up) by the reactions they catalyze.
Correct: This is not true. Enzymes are reused. - They are usually made of amino acids.
- They lower the activation energy of chemical reactions.
- Each one is specific to the particular substrate(s) to which it binds.
Why are enzymes so important to the function of cells?
Enzymes decrease the amount of energy required to start a chemical reaction. This makes it possible for our cells to build complex molecules with less energy investment than would otherwise be required.
Learn By Doing 5.12
Does physical exercise that increases muscle mass involve anabolic and/or catabolic processes? Give evidence for your answer.
Physical exercise involves both anabolic and catabolic processes. Body cells break down sugars to provide ATP to do the work necessary for exercise, such as muscle contractions. This is catabolism. Muscle cells also must repair muscle tissue damaged by exercise by building new muscle. This is anabolism.