Control and Regulation, An Introduction
13 Nervous System
Basic Organization of the Nervous System
Learning Objectives
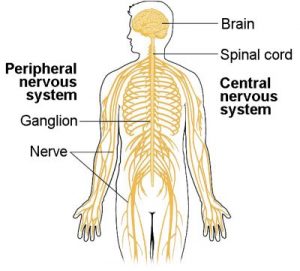
The nervous system is comprised of two major parts, or subdivisions, the central nervous system (CNS) and the peripheral nervous system (PNS). The CNS includes the brain and spinal cord. The brain is the body’s “control center.” The CNS has various centers located within it that carry out the sensory, motor, and integration of data. These centers can be subdivided into lower centers (including the spinal cord and brain stem) and higher centers communicating with the brain via effectors.
The PNS is a vast network of spinal and cranial nerves that are linked to the brain and the spinal cord. It contains sensory receptors which detect changes in the internal and external environment. This information is sent to the CNS via afferent sensory nerves. The PNS is then subdivided into two branches: the autonomic and the somatic nervous system. The autonomic branch has control of internal organs, blood vessels, and smooth and cardiac muscle. The somatic branch has control of skin, bones, joints, and skeletal muscle. The two systems function together by way of nerves from the PNS entering and becoming part of the CNS and vice versa.
We’ll further discuss the roles of these systems and their structures later in this chapter.
The nervous system has three main functions: sensory input, integration of data, and motor output. Sensory input is when the body gathers information or data by way of neurons, glia, and synapses. The nervous system is composed of excitable nerve cells (neurons) and support cells (glia). Specialized structures called synapses form between neurons. Synapses allow neurons to communicate with each other and to centers throughout the body. These neurons operate on excitation or inhibition, and although nerve cells can vary in size and location, their communication with one another determines their function. These nerves conduct impulses from sensory receptors to the brain and spinal cord. This information is then processed by way of integration of data, which occurs only in the brain. After the brain has processed the information, impulses are then conducted from the brain and spinal cord to muscles and glands, a process called motor output. Glia cells are found within tissues and are not excitable but are involved in myelination and the regulation of ions in extracellular fluid.
Learn By Doing 9.1
Which of the following is not a function of the nervous system?
- contribute to homeostatic feedback loops
- stimulate muscles and glands
- produce quick effects by electrochemical mechanisms
- release chemicals into the bloodstream for distribution throughout the body
Neurons and Glia
The nervous system of the fruit fly contains around 100,000 neurons, the same number as a lobster. This number compares to 75 million in the mouse and 300 million in the octopus. A human brain contains around 86 billion neurons. Despite these very different numbers, the nervous systems of these animals control many of the same behaviors—from basic reflexes to more complicated behaviors like finding food and courting mates. The ability of neurons to communicate with each other as well as with other types of cells underlies all of these behaviors.
Most neurons share the same cellular components. But neurons are also highly specialized—different types of neurons have different sizes and shapes that relate to their functional roles.
Parts of a Neuron
Like other cells, each neuron has a cell body (or soma) that contains a nucleus, smooth and rough endoplasmic reticulum, Golgi apparatus, mitochondria, and other cellular components. Neurons also contain unique structures, illustrated below, for receiving and sending the electrical signals that make neuronal communication possible. Dendrites are tree-like structures that extend away from the cell body and receive messages from other neurons at specialized junctions called synapses. Although some neurons do not have any dendrites, some types of neurons have multiple dendrites. Dendrites can have small protrusions called dendritic spines, which further increase surface area for possible synaptic connections.
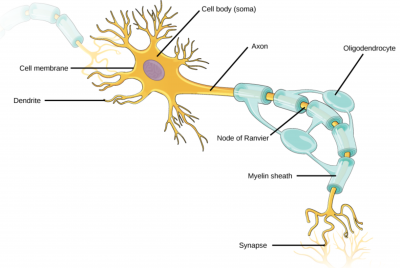
Once a signal is received by the dendrite, it then travels passively to the cell body. The cell body contains a specialized structure, the axon hillock that integrates signals from multiple synapses. This structure sits between the cell body and an axon. An axon is a tube-like structure that propagates the integrated signal to specialized endings called axon terminals. These terminals in turn synapse on other neurons, muscles, or target organs. Chemicals called neurotransmitters are released at axon terminals, allowing signals to be communicated to these other cells. Some axons are covered with myelin, which acts as an insulator to prevent loss of the electrical signal as it travels down the axon, greatly increasing the speed of conduction. This insulation is important, as the axon from a human motor neuron can be as long as a meter—from the base of the spine to the toes. The myelin sheath is not actually part of the neuron. Myelin is produced by glial cells. Along the axon, there are periodic gaps in the myelin sheath. These gaps are called nodes of Ranvier and are sites where the signal is “recharged” as it travels along the axon.
It is important to note that a single neuron does not act alone—neuronal communication depends on the connections that neurons make with one another (as well as with other cells, like muscle cells). Dendrites from a single neuron may receive synaptic contact from many other neurons. For example, dendrites from certain cells in the cerebellum are thought to receive contact from as many as 200,000 other neurons.
Learn By Doing 9.2
Below are questions about the relationship between parts of the neuron and their functions. To answer, choose from axon, axon hillock, cell body (soma), axon terminal, or dendrite.
- Which part of the neuron has plasma membrane proteins that will initiate an action potential a long distance away toward its target if the signal reaches a strong enough level called the threshold?
Hint: This is a funnel-shaped part of the cell where one part of the neuron connects to another part. - Which part of the neuron has plasma membrane proteins that convert stimuli to graded electrical potentials?
Hint: It is the part with increased surface area to allow better reception of this chemical message. - Which part of the neuron converts electrical potential to chemical stimuli for other neurons or target effectors?
Hint: This would happen at the “end” of the neuron that communicates with another neuron or its target across a space. - Which part of the neuron has most of the typical cellular organelles and the nucleus?
Hint: All parts have cytoplasm and some organelles, and material can move around within the cytoplasm, but one area has the nucleus and carries out the majority of cellular metabolism.
Types of Neurons
There are different types of neurons, and the functional role of a given neuron is intimately dependent on its structure. There is an amazing diversity of neuron shapes and sizes found in different parts of the nervous system (and across species), as illustrated by the neurons shown in the figure below.
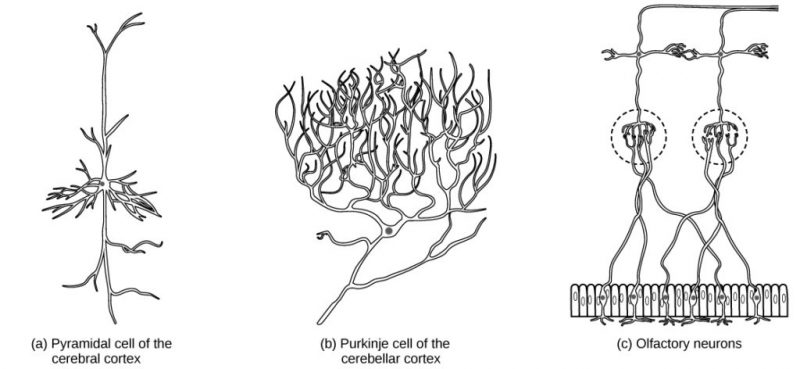
While there are many defined neuron cell subtypes, neurons are broadly divided into three basic types in mammals: bipolar, multipolar, and pseudounipolar. The figure below illustrates four basic neuron types. A bipolar neuron has one axon and one dendrite extending from the soma. An example of a bipolar neuron is a retinal bipolar cell.
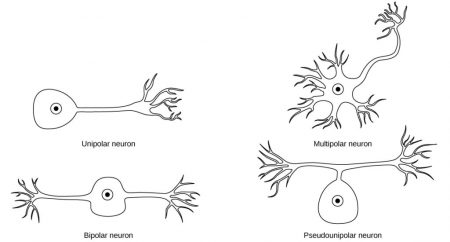
These cells receive signals from photoreceptor cells that are sensitive to light and transmit these signals to neurons that carry the signal to the brain. Multipolar neurons are the most common type of neuron. Each multipolar neuron contains one axon and multiple dendrites. Multipolar neurons can be found in the central nervous system (brain and spinal cord). An example of a multipolar neuron is a Purkinje cell in the cerebellum, which has many branching dendrites but only one axon. Unipolar neurons are not found in mammals. Pseudounipolar neurons share characteristics with both unipolar and bipolar cells. A pseudounipolar cell has a single process that extends from the soma, but this process later branches into two distinct structures, like a bipolar cell. Most sensory neurons are pseudounipolar and have an axon that branches into two extensions: one connected to dendrites that receive sensory information and another that transmits this information to the spinal cord.
Learn By Doing 9.3
The function of the whole nervous system is to receive input about changes in the internal and external environment, integrate and coordinate that input, and generate an output. This mirrors the components of homeostatic mechanisms. The single neuron (cell) is well suited because its different parts, dendrites, soma, and axon, serve these functions on a smaller scale.
- Which part of the neuron receives chemical input, either serving as or associated with a “receptor”?
- Which part of the neuron sums electrical impulses?
- Which part of the neuron sends output to the “effector”?
Neurogenesis
At one time, scientists believed that people were born with all the neurons they would ever have. Research performed during the last few decades indicates that neurogenesis, the birth of new neurons, continues into adulthood. Neurogenesis was first discovered in songbirds that produce new neurons while learning songs. For mammals, new neurons also play an important role in learning: about 1000 new neurons develop in the hippocampus (a brain structure involved in learning and memory) each day. While most of the new neurons will die, researchers found that an increase in the number of surviving new neurons in the hippocampus correlated with how well rats learned a new task. Interestingly, both exercise and some antidepressant medications also promote neurogenesis in the hippocampus. Stress has the opposite effect. While neurogenesis is quite limited compared to regeneration in other tissues, research in this area may lead to new treatments for disorders such as Alzheimer’s, stroke, and epilepsy.
Glial Cells
While glia are often thought of as the supporting cast of the nervous system, the number of glial cells in the brain actually outnumbers the number of neurons by a factor of ten. Neurons would be unable to function without the vital roles that are fulfilled by these glial cells. Glia guide developing neurons to their destinations, buffer ions and chemicals that would otherwise harm neurons and provide myelin sheaths around axons. Scientists have recently discovered that they also play a role in responding to nerve activity and modulating communication between nerve cells. When glia do not function properly, the result can be disastrous—mutations cause most brain tumors in glia.
Types of Glia
There are several different types of glia with different functions, which are shown below.
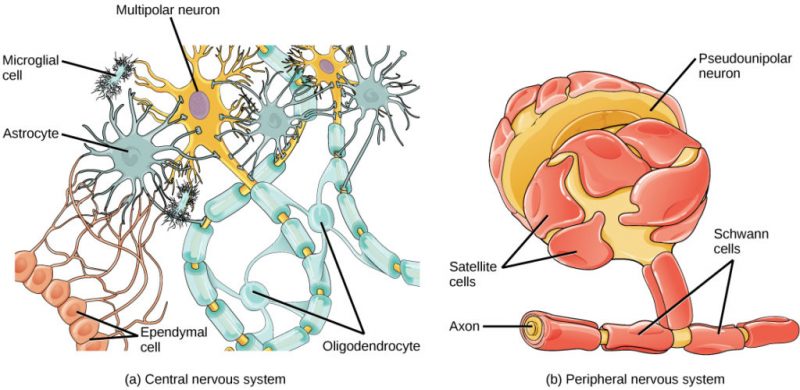
In the CNS, astrocytes make contact with both capillaries and neurons. They provide nutrients and other substances to neurons, regulate the concentrations of ions and chemicals in the extracellular fluid, and provide structural support for synapses. Astrocytes also form the blood-brain barrier—a structure that blocks the entrance of toxic substances into the brain. Microglia scavenge and degrade dead cells and protect the brain from invading microorganisms. Oligodendrocytes form myelin sheaths around axons in the CNS. One axon can be myelinated by several oligodendrocytes, and one oligodendrocyte can provide myelin for multiple neurons. Radial glia serve as scaffolds for developing neurons as they migrate to their end destinations. Ependymal cells line fluid-filled ventricles of the brain and the central canal of the spinal cord. They are involved in the production of cerebrospinal fluid, which serves as a cushion for the brain and moves the fluid between the spinal cord and the brain.
In the PNS, Satellite glia provide nutrients and structural support for neurons. The myelin sheaths around axons are provided by Schwann cells. Unlike the CNS, where many oligodendrocytes provide myelin to multiple axons, many individual Schwann cells myelinate one axon.
Learn By Doing 9.4
What type of glial cell is the resident macrophage within the brain?
- microglia
- astrocyte
- Schwann cell
- satellite cell
What type of glial cell provides myelin for the axons in a tract?
- oligodendrocyte
- astrocyte
- Schwann cell
- satellite cell
What types of macromolecule is the main component of myelin?
- carbohydrates
- proteins
- lipids
- nucleic acids
The main difference between oligodendrocytes and Schwann cells is…
- the type of myelin they produce
- whether one or multiple cells are required to myelinate any axon
- their location
- whether or not they insulate and facilitate signal propagation
Multiple sclerosis is a demyelinating disease affecting the central nervous system. What type of cell would be the most likely target of this disease? Why?
Communication Between Neurons
All functions performed by the nervous system—from a simple motor reflex to more advanced functions like making a memory or a decision—require neurons to communicate with one another. While humans use words and body language to communicate, neurons use electrical and chemical signals. Just like a person in a committee, one neuron usually receives and synthesizes messages from multiple other neurons before “making the decision” to send the message on to other neurons.
Learning Objectives
- Describe the basis of the resting membrane potential.
- Explain the stages of an action potential and how action potentials are propagated.
- Explain the structure and function of chemical synapses.
- Describe how information gathered by dendrite is integrated.
Resting Membrane Potential
For the nervous system to function, neurons must be able to send and receive signals. These signals are possible because each neuron has a charged cellular membrane (a voltage difference between the inside and the outside), and the charge of this membrane can change in response to neurotransmitter molecules released from other neurons and environmental stimuli. To understand how neurons communicate, one must first understand the basis of the baseline or “resting” membrane charge.
Neuronal Charged Membranes
The lipid bilayer membrane that surrounds a neuron is impermeable to charged molecules or ions. To enter or exit the neuron, ions must pass through special proteins called ion channels that span the membrane. Ion channels have different configurations: open, closed, and inactive, as illustrated below. Some ion channels need to be activated in order to open and allow ions to pass into or out of the cell. These ion channels are sensitive to the environment and can change their shape accordingly. Ion channels that change their structure in response to voltage changes are called voltage-gated ion channels. Voltage-gated ion channels regulate the relative concentrations of different ions inside and outside the cell. The difference in total charge between the inside and outside of the cell is called the membrane potential.
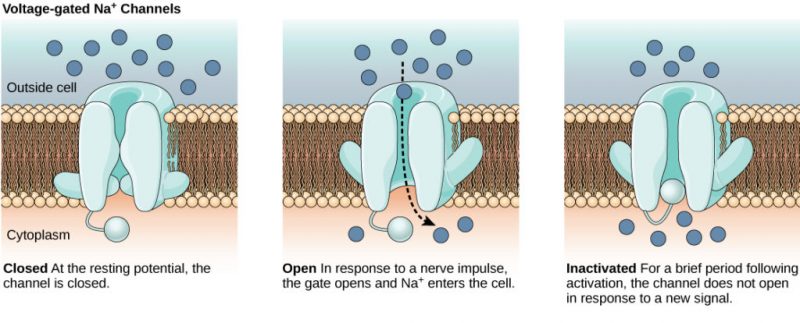
Resting Membrane Potential
A neuron at rest is negatively charged: the inside of a cell is approximately 70 millivolts more negative than the outside (−70 mV, note that this number varies by neuron type and by species). This voltage is called the resting membrane potential; it is caused by differences in the concentrations of ions inside and outside the cell. If the membrane were equally permeable to all ions, each type of ion would flow across the membrane and the system would reach equilibrium. Because ions cannot simply cross the membrane at will, there are different concentrations of several ions inside and outside the cell.
Ion Concentration Inside and Outside Neurons
The resting membrane potential is a result of different concentrations inside and outside the cell. The difference in the number of positively charged potassium ions (K+) inside and outside the cell dominates the resting membrane potential.
Ion | Extracellular concentration (mM) | Intracellular concentration (mM) | Ratio outside/inside |
Na+ | 145 | 12 | 12 |
K+ | 4 | 155 | 0.026 |
Cl− | 120 | 4 | 30 |
Organic anions (A−) | — | 100 |
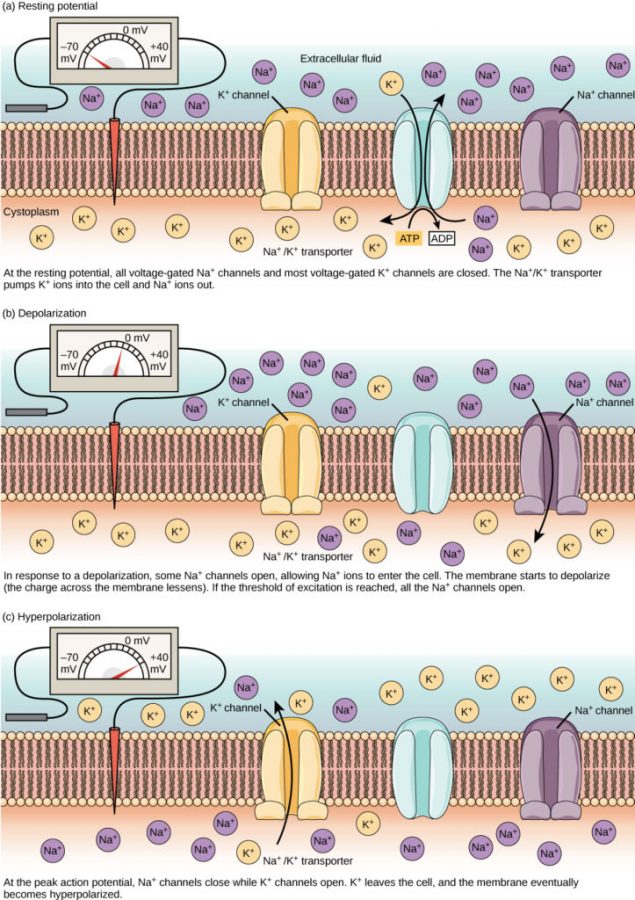
When the membrane is at rest, K+ ions accumulate inside the cell due to a net movement with the concentration gradient. The negative resting membrane potential is created and maintained by increasing the concentration of cations outside the cell (in the extracellular fluid) relative to inside the cell (in the cytoplasm). The negative charge within the cell is created by the cell membrane being more permeable to potassium ion movement than sodium ion movement. In neurons, potassium ions are maintained at high concentrations within the cell while sodium ions are maintained at high concentrations outside of the cell. The cell possesses potassium and sodium leakage channels that allow the two cations to diffuse down their concentration gradient.
However, the neurons have far more potassium leakage channels than sodium leakage channels. Therefore, potassium diffuses out of the cell at a much faster rate than sodium leaks in. Because more cations are leaving the cell than are entering, this causes the interior of the cell to be negatively charged relative to the outside of the cell. The actions of the sodium-potassium pump help to maintain the resting potential once established. Recall that sodium-potassium pumps bring two K+ ions into the cell while removing three Na+ ions per ATP consumed. As more cations are expelled from the cell than taken in, the inside of the cell remains negatively charged relative to the extracellular fluid. It should be noted that calcium ions (Ca2+) tend to accumulate outside of the cell because they are repelled by negatively-charged proteins within the cytoplasm.
Action Potential
A neuron can receive input from other neurons and, if this input is strong enough, send the signal to downstream neurons. Transmission of a signal between neurons is generally carried by a chemical called a neurotransmitter. Transmission of a signal within a neuron (from dendrite to axon terminal) is carried by a brief reversal of the resting membrane potential called an action potential. When neurotransmitter molecules bind to receptors located on a neuron’s dendrites, ion channels open. At excitatory synapses, this opening allows positive ions to enter the neuron and results in depolarization of the membrane—a decrease in the difference in voltage between the inside and outside of the neuron. A stimulus from a sensory cell or another neuron depolarizes the target neuron to its threshold potential (−55 mV). Na+ channels in the axon hillock open, allowing positive ions to enter the cell.
Once the sodium channels open, the neuron completely depolarizes to a membrane potential of about +40 mV. Action potentials are considered an “all-or-nothing” event because once the threshold potential is reached, the neuron always completely depolarizes. Once depolarization is complete, the cell must now “reset” its membrane voltage back to the resting potential. To accomplish this, the Na+ channels close and cannot be opened. This begins the neuron’s refractory period, in which it cannot produce another action potential because its sodium channels will not open. At the same time, voltage-gated K+ channels open, allowing K+ to leave the cell. As K+ ions leave the cell, the membrane potential once again becomes negative. The diffusion of K+ out of the cell actually hyperpolarizes the cell in that the membrane potential becomes more negative than the cell’s normal resting potential. At this point, the sodium channels will return to their resting state, meaning they are ready to open again if the membrane potential again exceeds the threshold potential. Eventually, the extra K+ ions diffuse out of the cell through the potassium leakage channels, bringing the cell from its hyperpolarized state back to its resting membrane potential.
The formation of an action potential can be divided into five steps, illustrated by the graph below.
- A stimulus from a sensory cell or another neuron causes the target cell to depolarize toward the threshold potential.
- If the threshold of excitation is reached, all Na+ channels open and the membrane depolarizes.
- At the peak action potential, K+ channels open and K+ begins to leave the cell. At the same time, Na+ channels close.
- The membrane becomes hyperpolarized as K+ ions continue to leave the cell. The hyperpolarized membrane is in a refractory period and cannot fire.
- The K+ channels close, and the Na+/K+ transporter restores the resting potential.
Once the action potential begins, it continues down the length of the axon until it reaches the axon terminals. Recall that this process, called impulse conduction (or propagation of the action potential) is referred to as “all or none” since it either happens or doesn’t.
Learn By Doing #5
The simulation below allows you to stimulate a neuron and monitor what happens. You can pause, rewind, and move forward in time in order to observe the ions as they move across the neuron membrane. As you explore the stimulation, consider these questions:
- What prevents ions from moving across neuron membranes?
- What allows ions to move across neuron membranes?
- What are gated channels? What are their functions?
- How does membrane permeability change because of voltage-gated channels?
- What is the sequence of events that generates an action potential?
Learn By Doing 9.6
What ion enters a neuron causing depolarization of the cell membrane?
- sodium
- chloride
- potassium
- phosphate
Voltage-gated Na+ channels open upon reaching what state?
- resting potential
- threshold
- repolarization
- overshoot
What does it mean for an action potential to be an “all or none” event?
Myelin and the Propagation of the Action Potential
For an action potential to communicate information to another neuron, it must travel along the axon and reach the axon terminals where it can initiate neurotransmitter release. The speed of conduction of an action potential along an axon is influenced by both the diameter of the axon and the axon’s resistance to current leak. Myelin acts as an insulator that prevents current from leaving the axon; this increases the speed of action potential conduction. In demyelinating diseases like multiple sclerosis, action potential conduction slows because current leaks from previously insulated axon areas.
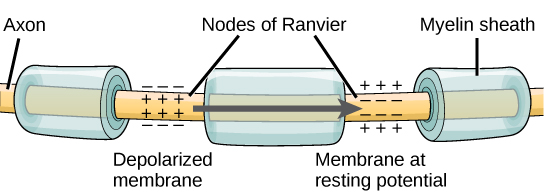
The Nodes of Ranvier, illustrated above, are gaps in the myelin sheath along the axon. These unmyelinated spaces are about one micrometer long and contain voltage-gated Na+ and K+ channels. The flow of ions through these channels, particularly the Na+ channels, regenerates the action potential over and over again along the axon. This ‘jumping’ of the action potential from one node to the next is called saltatory conduction. If Nodes of Ranvier were not present along an axon, the action potential would propagate very slowly since Na+ and K+ channels would have to continuously regenerate action potentials at every point along the axon instead of at specific points. Nodes of Ranvier also save energy for the neuron since the channels only need to be present at the nodes and not along the entire axon.
Learn By Doing 9.7
Which of the following is probably going to propagate an action potential fastest?
- a thin, unmyelinated axon
- a thin, myelinated axon
- a thick, unmyelinated axon
- a thick, myelinated axon
Chemical and Electrical Synapses
The synapse or “gap” is the place where information is transmitted from one neuron to another. Synapses usually form between axon terminals and dendritic spines, but this is not universally true. There are also axon-to-axon, dendrite-to-dendrite, and axon-to-cell body synapses. The neuron transmitting the signal is called the presynaptic neuron, and the neuron receiving the signal is called the postsynaptic neuron. Note that these designations are relative to a particular synapse—most neurons are both presynaptic and postsynaptic. There are two types of synapses: chemical and electrical.
Chemical Synapse
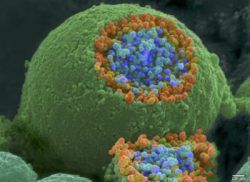
When an action potential reaches the axon terminal, it depolarizes the membrane and opens voltage-gated Na+ channels. Na+ ions enter the cell, further depolarizing the presynaptic membrane. This depolarization causes voltage-gated Ca2+ channels to open. Calcium ions entering the cell initiate a signaling cascade that causes small membrane-bound vesicles, called synaptic vesicles, containing neurotransmitter molecules to fuse with the presynaptic membrane. Synaptic vesicles are shown above in an image from a scanning electron microscope.
The fusion of a vesicle with the presynaptic membrane causes neurotransmitters to be released into the synaptic cleft, the extracellular space between the presynaptic and postsynaptic membranes, as illustrated below. The neurotransmitter diffuses across the synaptic cleft and binds to receptor proteins on the postsynaptic membrane.
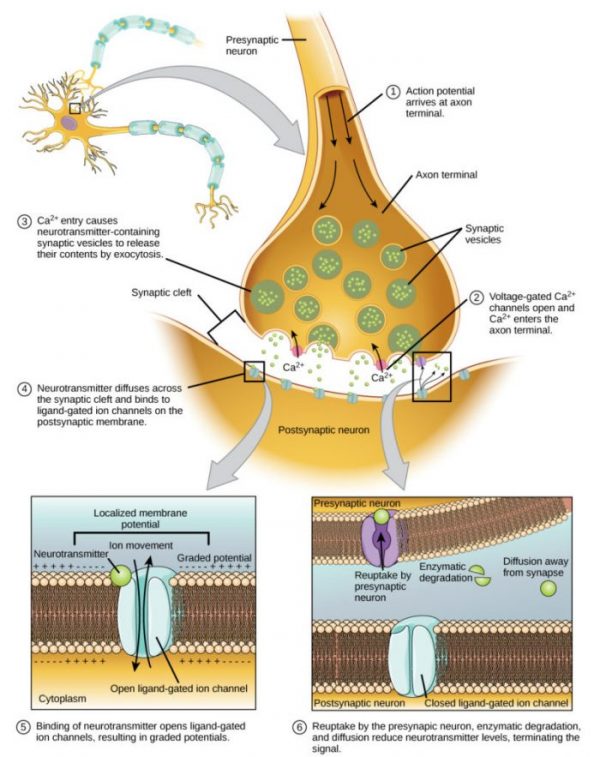
The binding of a specific neurotransmitter causes particular ion channels, in this case, ligand-gated channels, on the postsynaptic membrane to open. Neurotransmitters can either have excitatory or inhibitory effects on the postsynaptic membrane. There are several examples of well-known neurotransmitters detailed in the table below. For example, when acetylcholine is released at the synapse between a nerve and muscle (called the neuromuscular junction) by a presynaptic neuron, it causes postsynaptic Na+ channels to open. Na+ enters the postsynaptic cell and causes the postsynaptic membrane to depolarize. This depolarization is called an excitatory postsynaptic potential (EPSP) and makes the postsynaptic neuron more likely to fire an action potential. Release of neurotransmitters at inhibitory synapses causes inhibitory postsynaptic potentials (IPSPs), which are hyperpolarization of the presynaptic membrane. For example, when the neurotransmitter GABA (gamma-aminobutyric acid) is released from a presynaptic neuron, it binds to and opens Cl– channels. Cl– ions enter the cell and hyperpolarize the mem brane, making the neuron less likely to fire an action potential.
Neurotransmitter | Function | Location |
Acetylcholine | muscle control, memory | CNS and/or PNS |
Serotonin | intestinal movement, mood regulation, sleep | gut, CNS |
Dopamine | voluntary muscle movements, cognition, reward pathways | hypothalamus |
Norepinephrine | fight or flight response | adrenal medulla |
GABA | inhibits CNS | brain |
Glutamate | generally an excitatory neurotransmitter, memory | CNS, PNS |
Once neurotransmission has occurred, the neurotransmitter must be removed from the synaptic cleft so the postsynaptic membrane can “reset” and be ready to receive another signal. This can be accomplished in three ways: the neurotransmitter can diffuse away from the synaptic cleft, it can be degraded by enzymes in the synaptic cleft, or it can be recycled (sometimes called reuptake) by the presynaptic neuron. Several drugs act at this step of neurotransmission. For example, some drugs that are given to Alzheimer’s patients work by inhibiting acetylcholinesterase, the enzyme that degrades acetylcholine. This inhibition of the enzyme essentially increases neurotransmission at synapses that release acetylcholine. Once released, the acetylcholine stays in the cleft and can continually bind and unbind to postsynaptic receptors.
Electrical Synapse
While electrical synapses are fewer in number than chemical synapses, they are found in all nervous systems and play important and unique roles. The mode of neurotransmission in electrical synapses is quite different from that in chemical synapses. In an electrical synapse, the presynaptic and postsynaptic membranes are very close together and are actually physically connected by channel proteins forming gap junctions. Gap junctions allow current to pass directly from one cell to the next. In addition to the ions that carry this current, other molecules, such as ATP, can diffuse through the large gap junction pores.
There are key differences between chemical and electrical synapses. Because chemical synapses depend on the release of neurotransmitter molecules from synaptic vesicles to pass on their signal, there is an approximately one-millisecond delay between when the axon potential reaches the presynaptic terminal and when the neurotransmitter leads to the opening of postsynaptic ion channels. Additionally, this signaling is unidirectional. Signaling in electrical synapses, in contrast, is virtually instantaneous (which is important for synapses involved in key reflexes), and some electrical synapses are bidirectional. Electrical synapses are also more reliable as they are less likely to be blocked, and they are important for synchronizing the electrical activity of a group of neurons. For example, electrical synapses in the thalamus are thought to regulate slow-wave sleep, and disrupting these synapses can cause seizures.
Learn By Doing 9.8
Binding of a neurotransmitter to the post-synaptic membrane opens which type of channel?
- mechanically-gated
- ligand-gated
- voltage-gated
What happens to a postsynaptic cell membrane if chloride channels open as a result of neurotransmitter binding?
- The cell membrane depolarizes.
- IPSP (inhibitory postsynaptic potential) is achieved.
- EPSP (excitatory postsynaptic potential) occurs.
- The cell membrane repolarizes.
Hint: Negatively charged chloride ions are more concentrated outside the cell membrane and will follow their concentration gradient to diffuse across an open membrane channel.
Is the entry of calcium into the presynaptic axon terminus an active or passive process? Explain.
Would a spinal nerve have afferent or efferent transmission or both? Explain your reasoning.
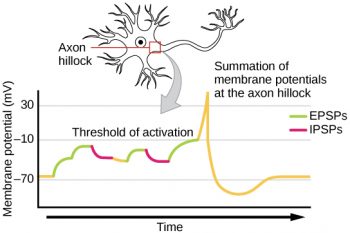
Integration of Information Within the Neuron
Sometimes a single EPSP is strong enough to induce an action potential in the postsynaptic neuron, but often multiple presynaptic inputs must create EPSPs around the same time for the postsynaptic neuron to be sufficiently depolarized to fire an action potential. This process is called signal summation and occurs at the axon hillock, as illustrated in Figure 9.8. Additionally, one neuron often has inputs from many presynaptic neurons—some excitatory and some inhibitory—so IPSPs can cancel out EPSPs and vice versa. It is the net change in postsynaptic membrane voltage that determines whether the postsynaptic cell has reached its threshold of excitation needed to fire an action potential. Together, synaptic summation and the threshold for excitation act as a filter so that random “noise” in the system is not transmitted as important information.
Example: Brain-Computer Interface
Amyotrophic lateral sclerosis (ALS, also called Lou Gehrig’s Disease) is a neurological disease characterized by the degeneration of the motor neurons that control voluntary movements. The disease begins with muscle weakening and lack of coordination and eventually destroys the neurons that control speech, breathing, and swallowing; in the end, the disease can lead to paralysis. At that point, patients require assistance from machines to be able to breathe and communicate. Several special technologies have been developed to allow “locked-in” patients to communicate with the rest of the world. One technology, for example, allows patients to type out sentences by twitching their cheeks. These sentences can then be read aloud by a computer.
A relatively new line of research for helping paralyzed patients, including those with ALS, to communicate and retain a degree of self-sufficiency is called brain-computer interface (BCI) technology and is illustrated in Figure 9.9. This technology sounds like something out of science fiction: it allows paralyzed patients to control a computer using only their thoughts. There are several forms of BCI. Some forms use EEG recordings from electrodes taped onto the skull. These recordings contain information from large populations of neurons that can be decoded by a computer. Other forms of BCI require the implantation of an array of electrodes smaller than a postage stamp in the arm and hand area of the motor cortex. This form of BCI, while more invasive, is very powerful as each electrode can record actual action potentials from one or more neurons. These signals are then sent to a computer, which has been trained to decode the signal and feed it to a tool—such as a cursor on a computer screen. This means that a patient with ALS can use e-mail, read the Internet, and communicate with others by thinking of moving his or her hand or arm (even though the paralyzed patient cannot make that bodily movement). Recent advances have allowed a paralyzed locked-in patient who suffered a stroke 15 years ago to control a robotic arm and even to feed herself coffee using BCI technology.
Despite the amazing advancements in BCI technology, it also has limitations. The technology can require many hours of training and long periods of intense concentration for the patient; it can also require brain surgery to implant the devices.
Synaptic Plasticity
Synapses are not static structures. They can be weakened or strengthened. They can be broken, and new synapses can be made. Synaptic plasticity allows for these changes, which are all needed for a functioning nervous system. In fact, synaptic plasticity is the basis of learning and memory. Two processes in particular, long-term potentiation (LTP) and long-term depression (LTD) are important forms of synaptic plasticity that occur in synapses in the hippocampus, a brain region that is involved in storing memories.
Learn By Doing 9.9
Which of the following is true only about action potentials but not about graded potentials?
- inhibitory or excitatory
- can decrease in strength over time
- graded
- uses voltage-gated channels
- reversible
Hint: Graded potentials are also known as local potentials that can initiate the propagation of an action potential that travels one direction from the trigger zone to the axon terminal very quickly.
Why should the opening of a K+ channel cause hyperpolarization?
Hint: what are the relative intracellular and extracellular concentrations of potassium?
Both graded potentials and action potentials can involve Na+ and K+ channels. What is different in how these channels behave that creates the different types of potentials?
The channels responsible for EPSPs are sodium channels, while those responsible for IPSPs are potassium and chloride channels. Can you explain why?
The Central Nervous System
The central nervous system contains the brain and the spinal cord, which are covered and protected by three layers of meninges. The brain contains structurally and functionally defined regions. While functions may be primarily localized to one structure in the brain, most complex functions, like language and sleep, involve neurons in multiple brain regions. The spinal cord is the information superhighway that connects the brain with the rest of the body through its connections with peripheral nerves. It transmits sensory and motor input and also controls motor reflexes.
Learning Objectives
- Identify the components of the central nervous system.
- Identify locations and basic functions of the different parts of the brain.
- Identify locations and basic functions of the different parts of the spinal cord.
Components of the Central Nervous System
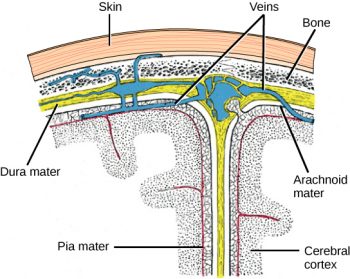
The central nervous system (CNS) is made up of the brain and spinal cord. Part of the skull and brain are shown in Figure 9.1. Note that the brain is covered with three layers of protective coverings called meninges (from the Greek word for membrane). The outermost layer is the dura mater (Latin for “hard mother”). As the Latin suggests, the primary function of this thick layer is to protect the brain and spinal cord. The dura mater also contains vein-like structures that carry blood from the brain back to the heart. The middle layer is the web-like arachnoid mater. The last layer is the pia mater (Latin for “soft mother”), which directly contacts and covers the brain and spinal cord like plastic wrap. The space between the arachnoid and pia maters is filled with cerebrospinal fluid (CSF). CSF is produced by a tissue called choroid plexus in fluid-filled compartments in the CNS called ventricles. The brain floats in CSF, which acts as a cushion and shock absorber and makes the brain neutrally buoyant. CSF also functions to circulate chemical substances throughout the brain and into the spinal cord.
The entire brain contains only about 8.5 tablespoons of CSF, but CSF is constantly produced in the ventricles. This creates a problem when a ventricle is blocked—the CSF builds up and creates swelling, and the brain is pushed against the skull. This swelling condition is called hydrocephalus (“water head”) and can cause seizures, cognitive problems, and even death if a shunt is not inserted to remove the fluid and pressure.
Learn By Doing 9.10
In addition to meninges, fluid-filled spaces help protect the brain’s nervous tissue. The subarachnoid space would be found between which two meninges?
- dura mater and arachnoid mater
- arachnoid and pia mater
- dura mater and pia mater
Brain
The brain is the part of the central nervous system that is contained in the cranial cavity of the skull. Anatomically, it includes the cerebrum, containing the cerebral cortex; the diencephalon, containing the basal ganglia, thalamus, and hypothalamus; the cerebellum; and the brainstem. There are also functional subdivisions of the brain, such as the limbic system.
There are three different ways that a brain can be sectioned in order to view internal structures: a sagittal section cuts the brain left to right, as shown on the right in the image below, a coronal section cuts the brain front to back, as shown on the left below, and a horizontal section cuts the brain top to bottom.
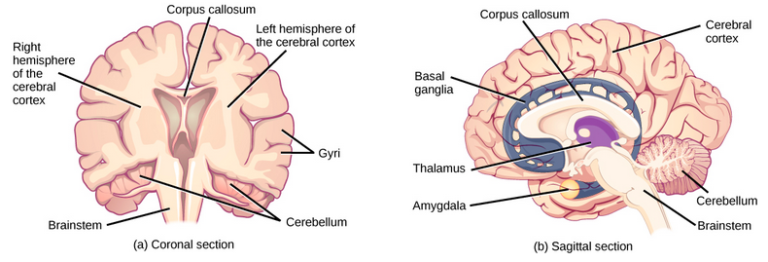
Cerebral Cortex
The outermost part of the brain is a thick piece of nervous system tissue called the cerebral cortex, which is folded into hills called gyri (singular: gyrus) and valleys called sulci (singular: sulcus). The cortex is made up of two hemispheres—right and left—which are separated by a large sulcus. A thick fiber bundle called the corpus callosum (Latin: “tough body”) connects the two hemispheres and allows information to be passed from one side to the other. Although there are some brain functions that are localized more to one hemisphere than the other, the functions of the two hemispheres are largely redundant. In fact, sometimes (very rarely) an entire hemisphere is removed to treat severe epilepsy. While patients do suffer some deficits following the surgery, they can have surprisingly few problems, especially when the surgery is performed on children who have very immature nervous systems.
In other surgeries to treat severe epilepsy, the corpus callosum is cut instead of removing an entire hemisphere. This causes a condition called split brain, which gives insights into the unique functions of the two hemispheres. For example, when an object is presented to patients’ left visual field, they may be unable to verbally name the object (and may claim not to have seen an object at all). This is because the visual input from the left visual field crosses and enters the right hemisphere and cannot then signal to the speech center, which generally is found on the left side of the brain. Remarkably, if a split-brain patient is asked to pick up a specific object out of a group of objects with the left hand, the patient will be able to do so but will still be unable to vocally identify it.
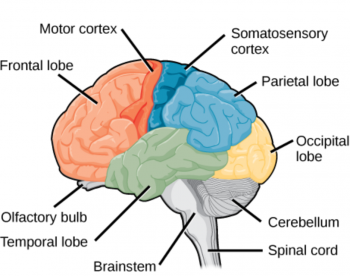
Each cortical hemisphere contains regions called lobes that are involved in different functions. Scientists use various techniques to determine what brain areas are involved in different functions: they examine patients who have had injuries or diseases that affect specific areas and see how those areas are related to functional deficits. They also conduct animal studies where they stimulate brain areas and see if there are any behavioral changes. They use a technique called trans-magnetic stimulation (TMS) to temporarily deactivate specific parts of the cortex using strong magnets placed outside the head, and they use functional magnetic resonance imaging (fMRI) to look at changes in oxygenated blood flow in particular brain regions that correlate with specific behavioral tasks. These techniques, and others, have given great insight into the functions of different brain regions but have also shown that any given brain area can be involved in more than one behavior or process, and any given behavior or process generally involves neurons in multiple brain areas. That being said, each hemisphere of the mammalian cerebral cortex can be broken down into four functionally and spatially defined lobes: frontal, parietal, temporal, and occipital. The figure above illustrates the four lobes of the human cerebral cortex.
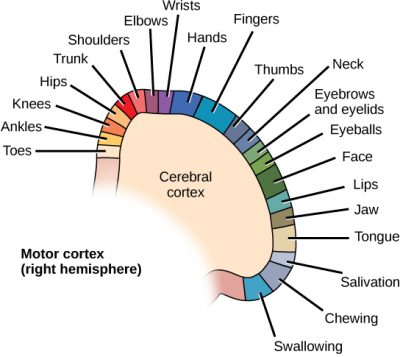
The frontal lobe is located at the front of the brain, over the eyes. This lobe contains the olfactory bulb, which processes smells. The frontal lobe also contains the motor cortex, which is important for planning and implementing movement. Areas within the motor cortex map to different muscle groups, and there is some organization to this map, as shown above. For example, the neurons that control movement of the fingers are next to the neurons that control movement of the hand. Neurons in the frontal lobe also control cognitive functions like maintaining attention, speech, and decision-making. Studies of humans who have damaged their frontal lobes show that parts of this area are involved in personality, socialization, and assessing risk.
The parietal lobe is located at the top of the brain. Neurons in the parietal lobe are involved in speech and also reading. Two of the parietal lobe’s main functions are processing somatosensation—touch sensations like pressure, pain, heat, and cold—and processing proprioception—the sense of how parts of the body are oriented in space. The parietal lobe contains a somatosensory map of the body similar to the motor cortex.
The occipital lobe is located at the back of the brain. It is primarily involved in vision—seeing, recognizing, and identifying the visual world.
The temporal lobe is located at the base of the brain by your ears and is primarily involved in processing and interpreting sounds. It also contains the hippocampus (Greek for “seahorse”)—a structure involved in memory formation. The role of the hippocampus in memory was partially determined by studying one famous epileptic patient, HM, who had both sides of his hippocampus removed in an attempt to cure his epilepsy. His seizures went away, but he could no longer form new memories (although he could remember some facts from before his surgery and could learn new motor tasks).
Cerebral Cortex
Compared to other vertebrates, mammals have exceptionally large brains for their body size. An entire alligator’s brain, for example, would fill about one and a half teaspoons. This increase in brain-to-body size ratio is especially pronounced in apes, whales, and dolphins. While this increase in overall brain size doubtlessly played a role in the evolution of complex behaviors unique to mammals, it does not tell the whole story. Scientists have found a relationship between the relatively high surface area of the cortex and the intelligence and complex social behaviors exhibited by some mammals. This increased surface area is due, in part, to the increased folding of the cortical sheet (more sulci and gyri). For example, a rat cortex is very smooth with very few sulci and gyri. Cat and sheep cortices have more sulci and gyri. Chimps, humans, and dolphins have even more.
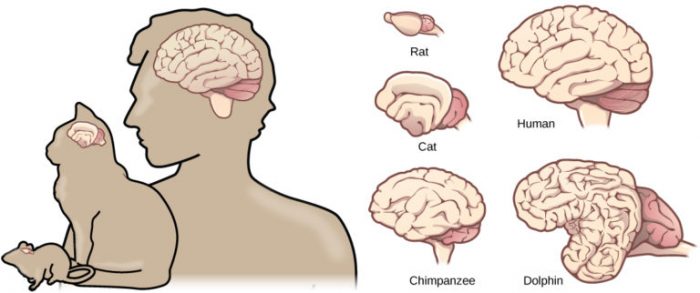
Learn By Doing 9.11
Which part of the brain has noticeable gyri and sulci that increase surface area for cortical gray matter?
- pons
- brainstem
- diencephalon
- left and right hemispheres
Which statement is true of grey matter?
- It is the primary source of intelligence
- It is located mainly in the frontal lobes
- It processes information
- It is the dominant type of nervous tissue in the brain
Which structure predominates in the white matter of the brain?
- myelinated axons
- neuronal cell bodies
- ganglia of the parasympathetic nerves
- bundles of dendrites from the enteric nervous system
Which area of the brain is not part of the cerebral cortex?
- Frontal lobe
- Cerebellum
- Parietal lobe
- Temporal lobe
Which part of the cerebral cortex contains the visual center?
- temporal lobe
- frontal lobe
- parietal lobe
- occipital lobe
Diencephalon
Basal Ganglia
Interconnected brain areas called the basal ganglia (or basal nuclei) play important roles in movement control and posture. Damage to the basal ganglia, as in Parkinson’s disease, leads to motor impairments like a shuffling gait when walking. The basal ganglia also regulate motivation. For example, when a wasp sting led to bilateral basal ganglia damage in a 25-year-old businessman, he began to spend all his days in bed and showed no interest in anything or anybody. But when he was externally stimulated—as when someone asked to play a card game with him—he was able to function normally. Interestingly, he and other similar patients do not report feeling bored or frustrated by their state.
Thalamus
The thalamus (Greek for “inner chamber”) acts as a gateway to and from the cortex. It receives sensory, and motor inputs from the body and also receives feedback from the cortex. This feedback mechanism can modulate conscious awareness of sensory and motor inputs depending on the attention and arousal state of the individual. The thalamus helps regulate consciousness, arousal, and sleep states. A rare genetic disorder called fatal familial insomnia causes the degeneration of thalamic neurons and glia. This disorder prevents affected patients from being able to sleep, among other symptoms, and is eventually fatal.
Hypothalamus
Below the thalamus is the hypothalamus. The hypothalamus controls the endocrine system by sending signals to the pituitary gland, a pea-sized endocrine gland that releases several different hormones that affect other glands as well as other cells. This relationship means that the hypothalamus regulates important behaviors that are controlled by these hormones. The hypothalamus is the body’s thermostat—it makes sure key functions like food and water intake, energy expenditure, and body temperature are kept at appropriate levels. Neurons within the hypothalamus also regulate circadian rhythms, sometimes called sleep cycles.
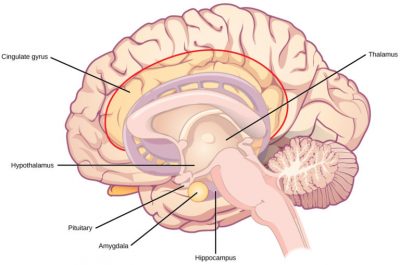
Limbic System
The limbic system is a connected set of structures that regulate emotion, as well as behaviors related to fear and motivation. It plays a role in memory formation and includes parts of the thalamus and hypothalamus as well as the hippocampus. One important structure within the limbic system is a temporal lobe structure called the amygdala (Greek for “almond”). The two amygdalas are important both for the sensation of fear and for recognizing fearful faces. The cingulate gyrus helps regulate emotions and pain.
Cerebellum
The cerebellum (Latin for “little brain”) sits at the base of the brain on top of the brainstem. The cerebellum controls balance and aids in coordinating movement and learning new motor tasks.
Brainstem
The brainstem connects the rest of the brain with the spinal cord. It consists of the midbrain, medulla oblongata, and pons. Motor and sensory neurons extend through the brainstem allowing for the relay of signals between the brain and the spinal cord. Ascending neural pathways cross in this section of the brain allowing the left hemisphere of the cerebrum to control the right side of the body and vice versa. The brainstem coordinates motor control signals sent from the brain to the body. The brainstem controls several important functions of the body including alertness, arousal, breathing, blood pressure, digestion, heart rate, swallowing, walking, and sensory and motor information integration.
Learn By Doing 9.12
Which part of the brain includes the hippocampus and amygdala and is involved in emotions and memory?
- precentral cerebral gyrus
- reticular activating formation
- postcentral cerebral gyrus
- limbic system
Spinal Cord
Connecting to the brainstem and extending down the body through the spinal column is the spinal cord. The spinal cord is a thick bundle of nerve tissue that carries information about the body to the brain and from the brain to the body. The spinal cord is contained within the bones of the vertebrate column but is able to communicate signals to and from the body through its connections with spinal nerves (part of the peripheral nervous system). A cross-section of the spinal cord looks like a white oval containing a gray butterfly shape. Myelinated axons make up the “white matter,” and neuron and glial cell bodies make up the “gray matter.” Gray matter is also composed of interneurons, which connect two neurons each located in different parts of the body. Axons and cell bodies in the dorsal (facing the back of the animal) spinal cord convey mostly sensory information from the body to the brain. Axons and cell bodies in the ventral (facing the front of the animal) spinal cord primarily transmit signals controlling movement from the brain to the body.
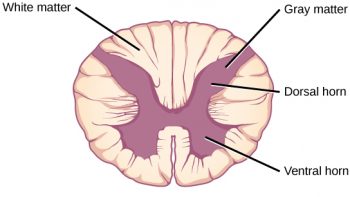
The spinal cord also controls motor reflexes. These reflexes are quick, unconscious movements—like automatically removing a hand from a hot object. Reflexes are so fast because they involve local synaptic connections. For example, the knee reflex that a doctor tests during a routine physical is controlled by a single synapse between a sensory neuron and a motor neuron. While a reflex may only require the involvement of one or two synapses, synapses with interneurons in the spinal column transmit information to the brain to convey what happened (the knee jerked, or the hand was hot).
In the United States, there are around 10,000 spinal cord injuries each year. Because the spinal cord is the information superhighway connecting the brain with the body, damage to the spinal cord can lead to paralysis. The extent of the paralysis depends on the location of the injury along the spinal cord and whether the spinal cord was completely severed. For example, if the spinal cord is damaged at the level of the neck, it can cause paralysis from the neck down, whereas damage to the spinal column further down may limit paralysis to the legs. Spinal cord injuries are notoriously difficult to treat because spinal nerves do not regenerate, although ongoing research suggests that stem cell transplants may be able to act as a bridge to reconnect severed nerves. Researchers are also looking at ways to prevent the inflammation that worsens nerve damage after injury. One such treatment is to pump the body with cold saline to induce hypothermia. This cooling can prevent swelling and other processes that are thought to worsen spinal cord injuries.
Learn By Doing 9.13
Sensory fibers, or pathways, are referred to as “afferent.” Motor fibers, or pathways, are referred to as “efferent.” What can you infer about the meaning of these two terms (afferent and efferent) in a structural or anatomical context?
Would a spinal nerve have afferent or efferent transmission or both? Explain your reasoning.
The Peripheral Nervous System
Learning Objectives
- Describe the organization and functions of the sympathetic nervous systems.
- Describe the organization and functions of the parasympathetic nervous systems.
- Describe the organization and function of the sensory-somatic nervous system.
- Describe a spinal reflex arc.
The peripheral nervous system (PNS) is the connection between the central nervous system and the rest of the body. The CNS is like the power plant of the nervous system. It creates the signals that control the functions of the body. The PNS is like the wires that go to individual houses. Without those “wires,” the signals produced by the CNS could not control the body (and the CNS would not be able to receive sensory information from the body either).
The PNS can be broken down into the autonomic nervous system, which controls bodily functions without conscious control, and the sensory-somatic nervous system, which transmits sensory information from the skin, muscles, and sensory organs to the CNS and sends motor commands from the CNS to the muscles.
Autonomic Nervous System
In the autonomic nervous system, a preganglionic neuron of the CNS synapses with a postganglionic neuron of the PNS. The postganglionic neuron, in turn, acts on a target organ. Autonomic responses are mediated by the sympathetic and the parasympathetic systems, which are antagonistic to one another. The sympathetic system activates the “fight or flight” response, while the parasympathetic system activates the “rest and digest” response.
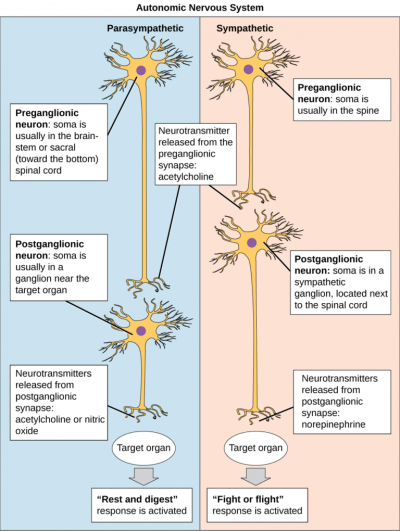
The autonomic nervous system serves as the relay between the CNS and the internal organs. It controls the lungs, the heart, smooth muscle, and exocrine and endocrine glands. The autonomic nervous system controls these organs largely without conscious control; it can continuously monitor the conditions of these different systems and implement changes as needed. Signaling to the target tissue usually involves two synapses: a preganglionic neuron (originating in the CNS) synapses to a neuron in a ganglion that, in turn, synapses on the target organ, as illustrated above. There are two divisions of the autonomic nervous system that often have opposing effects: the sympathetic nervous system and the parasympathetic nervous system.
Sympathetic Nervous System
The sympathetic nervous system is responsible for the “fight or flight” response that occurs when an animal encounters a dangerous situation. One way to remember this is to think of the surprise a person feels when encountering a snake (“snake” and “sympathetic” both begin with “s”). Examples of functions controlled by the sympathetic nervous system include an accelerated heart rate and inhibited digestion. These functions help prepare an organism’s body for the physical strain required to escape a potentially dangerous situation or to fend off a predator.
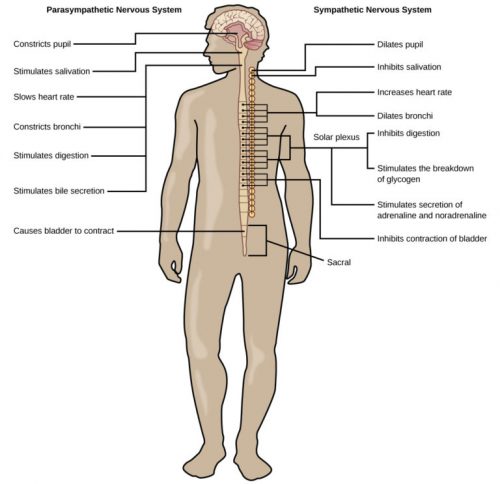
Parasympathetic Nervous System
While the sympathetic nervous system is activated in stressful situations, the parasympathetic nervous system allows an animal to “rest and digest.” The parasympathetic system’s functions conserve energy: slowing down the heart rate and reducing contractile forces of both cardiac and gastrointestinal muscles.
One way to remember this is to think that during a restful situation like a picnic, the parasympathetic nervous system is in control (“picnic” and “parasympathetic” both start with “p”). Parasympathetic preganglionic neurons have cell bodies located in the brainstem and in the sacral (toward the bottom) spinal cord. The parasympathetic nervous system resets organ function after the sympathetic nervous system is activated.
Sensory-Somatic Nervous System
The sensory-somatic nervous system is made up of cranial and spinal nerves and contains both sensory and motor neurons. Sensory neurons transmit sensory information from the skin, skeletal muscle, and sensory organs to the CNS. Motor neurons transmit messages about desired movement from the CNS to the muscles to make them contract. Without its sensory-somatic nervous system, an animal would be unable to process any information about its environment (what it sees, feels, hears, and so on) and could not control motor movements. Unlike the autonomic nervous system, which has two synapses between the CNS and the target organ, sensory and motor neurons have only one synapse—one ending of the neuron is at the organ, and the other directly contacts a CNS neuron. Acetylcholine is the main neurotransmitter released at these synapses.
Humans have 12 cranial nerves, nerves that emerge from or enter the skull (cranium), as opposed to the spinal nerves, which emerge from the vertebral column. Each cranial nerve has a name.
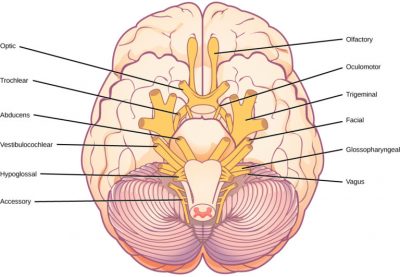
Some cranial nerves transmit only sensory information. For example, the olfactory nerve transmits information about smells from the nose to the brainstem. Other cranial nerves transmit almost solely motor information. For example, the oculomotor nerve controls the opening and closing of the eyelid and some eye movements. Other cranial nerves contain a mix of sensory and motor fibers. For example, the glossopharyngeal nerve has a role in both taste (sensory) and swallowing (motor).
Spinal nerves transmit sensory and motor information between the spinal cord and the rest of the body. Each of the 31 spinal nerves in humans contains both sensory and motor axons. The sensory neuron cell bodies are grouped in structures called dorsal root ganglia(singular: ganglion) as shown below.
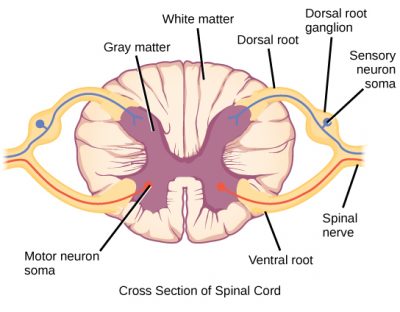
Each sensory neuron has one projection—with a sensory receptor ending in skin, muscle, or sensory organs—and another that synapses with a neuron in the dorsal spinal cord. Motor neurons have cell bodies in the ventral gray matter of the spinal cord that project to muscle through the ventral root. These neurons are usually stimulated by interneurons within the spinal cord but are sometimes directly stimulated by sensory neurons.
Spinal Reflexes
A reflex is a rapid automatic response to a stimulus. When you accidentally touch a hot object and automatically jerk your hand away, this is a reflex action. It happens without you having to think about it.
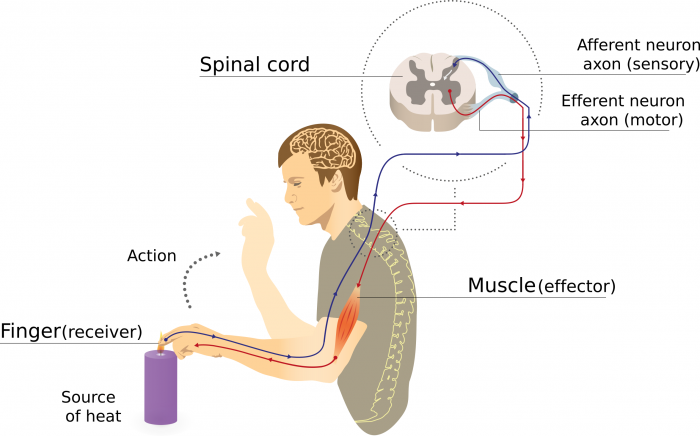
The path taken by the nerve impulses in a reflex is called a reflex arc. Most reflex arcs involve only three neurons as shown in the figure above. The stimulus, in this case, heat, stimulates the pain receptors of the skin, which initiate an action potential in a sensory neuron. This impulse travels to the spinal cord where it passes, by means of a synapse, to an interneuron (called the relay neuron) situated in the dorsal part of the grey matter. The relay neuron in turn makes a synapse with one or more motor neurons, stimulating an action potential. This leads to muscle contraction and the removal of the hand from the painful stimulus.
Reflexes do not require the involvement of the brain. although the information is transmitted there. Because the action is initiated without the brain’s involvement, the response occurs much faster than it otherwise would.
Learn By Doing 9.14
Each of the following neurons (cells) is part of the nervous system. Based on the description of its location and function, classify each as belonging to either the Central Nervous System division (CNS) or the Peripheral Nervous System division (PNS):
- Neuron is associated with thermoreceptor in skin.
- Neuron is associated with nasal chemoreceptors.
- Neuron is associated with internal thermoreceptors near hypothalamus.
- Neuron is located in the outer cortex of the brain, getting sensory input and initiating the voluntary override that prevented you from dropping the pan.
- Neuron is located in the hypothalamus, receiving input and initiating response to hypothermia or hyperthermia.
- Neuron is located in the spinal cord, transferring sensory input to motor output for reflex, such as the withdrawal reflex when finger touched hot pan.
- Neuron is carrying motor stimulus to arm muscle to pull away from hot pan.
- Neuron is carrying motor stimulus to arm muscle, overriding reflex to hold onto the pan a little longer.
- Neuron is carrying motor stimulus to sweat gland in skin to stimulate sweating and cool off body.
- Neuron is carrying motor stimulus to skeletal muscles to stimulate shivering to warm body.
Which of the following is not associated with the Peripheral Nervous System?
- motor output to skeletal muscles
- motor output to glands, smooth muscle and cardiac muscle
- integration of sensory input and initiation of voluntary or involuntary output
- sensory input from peripheral receptors
The fight-or-flight response is controlled by the…
- autonomic nervous system
- somatic nervous system
- fast nervous system
- sympathetic nervous system
For each of the statements below, decide which branch of the autonomic nervous system is likely to be involved.
- Faster blood flow and more blood flow to skeletal muscles
- Stimulation of sweat glands to reduce excessive body heat
- A good internal body state sitting down to a hearty meal
Nervous System: Differences in Function and Disorders
Learning Objectives
- Identify key characteristics and symptoms of neurodegenerative disorders
- Identify key characteristics and symptoms of neurodevelopmental disorders
- Identify key characteristics and symptoms of mental illnesses
- Identify key characteristics and symptoms of other neurological disorders
A nervous system that functions correctly is a fantastically complex, well-oiled machine—synapses fire appropriately, muscles move when needed, memories are formed and stored, and emotions are well-regulated. Unfortunately, each year millions of people in the United States deal with a nervous system disorder. While scientists have discovered potential causes of many of these diseases and viable treatments for some, ongoing research seeks to find ways to better prevent and treat all of these disorders.
Neurodegenerative Disorders
Neurodegenerative disorders are illnesses characterized by a loss of nervous system functioning that are usually caused by neuronal death. These diseases generally worsen over time as more and more neurons die. The symptoms of a particular neurodegenerative disease are related to where in the nervous system the death of neurons occurs. Spinocerebellar ataxia, for example, leads to neuronal death in the cerebellum. The death of these neurons causes problems in balance and walking. Neurodegenerative disorders include Huntington’s disease, amyotrophic lateral sclerosis, Alzheimer’s disease and other types of dementia disorders, and Parkinson’s disease. Here, Alzheimer’s and Parkinson’s disease will be discussed in more depth.
Alzheimer’s Disease
Alzheimer’s disease is the most common cause of dementia in the elderly. In 2012, an estimated 5.4 million Americans suffered from Alzheimer’s disease, and payments for their care are estimated at $200 billion. Roughly one in every eight people age 65 or older has the disease. Due to the aging of the baby-boomer generation, there are projected to be as many as 13 million Alzheimer’s patients in the United States in the year 2050.
Symptoms of Alzheimer’s Disease include disruptive memory loss, confusion about time or place, difficulty planning or executing tasks, poor judgment, and personality changes. Problems smelling certain scents can also be indicative of Alzheimer’s disease and may serve as an early warning sign. Many of these symptoms are also common in people who are aging normally, so it is the severity and longevity of the symptoms that determine whether a person is suffering from Alzheimer’s.
Alzheimer’s disease was named for Alois Alzheimer, a German psychiatrist who published a report in 1911 about a woman who showed severe dementia symptoms. Along with his colleagues, he examined the woman’s brain following her death and reported the presence of abnormal clumps, which are now called amyloid plaques, along with tangled brain fibers called neurofibrillary tangles. Amyloid plaques, neurofibrillary tangles, and an overall shrinking of brain volume are commonly seen in the brains of Alzheimer’s patients. Loss of neurons in the hippocampus is especially severe in advanced Alzheimer’s patients. Figure 1 compares a normal brain to the brain of an Alzheimer’s patient. Many research groups are examining the causes of these hallmarks of the disease.
One form of the disease is usually caused by mutations in one of three known genes. This rare form of early-onset Alzheimer’s disease affects fewer than five percent of patients with the disease and causes dementia beginning between the ages of 30 and 60. The more prevalent, late-onset form of the disease likely also has a genetic component. One particular gene, apolipoprotein E (APOE) has a variant (E4) that increases a carrier’s likelihood of getting the disease. Many other genes have been identified that might be involved in the pathology.
Unfortunately, there is no cure for Alzheimer’s disease. Current treatments focus on managing the symptoms of the disease. Because a decrease in the activity of cholinergic neurons (neurons that use the neurotransmitter acetylcholine) is common in Alzheimer’s disease, several drugs used to treat the disease work by increasing acetylcholine neurotransmission, often by inhibiting the enzyme that breaks down acetylcholine in the synaptic cleft. Other clinical interventions focus on behavioral therapies like psychotherapy, sensory therapy, and cognitive exercises. Since Alzheimer’s disease appears to hijack the normal aging process, research into prevention is prevalent. Smoking, obesity, and cardiovascular problems may be risk factors for the disease, so treatments for those may also help to prevent Alzheimer’s disease. Some studies have shown that people who remain intellectually active by playing games, reading, playing musical instruments, and being socially active in later life have a reduced risk of developing the disease.
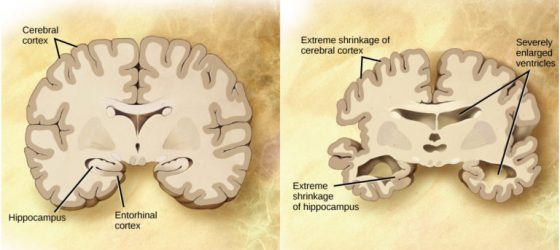
Parkinson’s Disease
Like Alzheimer’s disease, Parkinson’s Disease is a neurodegenerative disease. It was first characterized by James Parkinson in 1817. Each year, 50,000-60,000 people in the United States are diagnosed with the disease. Parkinson’s disease causes the loss of dopamine neurons in the substantia nigra, a midbrain structure that regulates movement. Loss of these neurons causes many symptoms including tremors (shaking of fingers or a limb), slowed movement, speech changes, balance and posture problems, and rigid muscles. The combination of these symptoms often causes a characteristic slow hunched shuffling walk, illustrated below. Patients with Parkinson’s disease can also exhibit psychological symptoms, such as dementia or emotional problems.
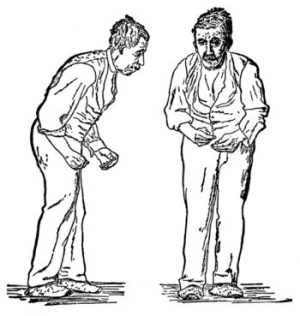
Although some patients have a form of the disease known to be caused by a single mutation, for most patients the exact causes of Parkinson’s disease remain unknown: the disease likely results from a combination of genetic and environmental factors (similar to Alzheimer’s disease). Post-mortem analysis of the brains of Parkinson’s patients shows the presence of Lewy bodies—abnormal protein clumps—in dopaminergic neurons. The prevalence of these Lewy bodies often correlates with the severity of the disease.
There is no cure for Parkinson’s disease, and treatment is focused on easing symptoms. One of the most commonly prescribed drugs for Parkinson’s is L-DOPA, which is a chemical that is converted into dopamine by neurons in the brain. This conversion increases the overall level of dopamine neurotransmission and can help compensate for the loss of dopaminergic neurons in the substantia nigra. Other drugs work by inhibiting the enzyme that breaks down dopamine.
Neurodevelopmental Disorders
Neurodevelopmental disorders occur when the development of the nervous system is disturbed. There are several different classes of neurodevelopmental disorders. Some, like Down Syndrome, cause intellectual deficits. Others specifically affect communication, learning, or the motor system. Some disorders like autism spectrum disorder and attention-deficit/hyperactivity disorder have complex symptoms.
Autism
Autism spectrum disorder (ASD) is a neurodevelopmental disorder. Its severity differs from person to person. Estimates for the prevalence of the disorder have changed rapidly in the past few decades. Current estimates suggest that one in 88 children will develop the disorder. ASD is four times more prevalent in males than females.
A characteristic symptom of ASD is impaired social skills. Children with autism may have difficulty making and maintaining eye contact and reading social cues. They also may have problems feeling empathy for others. Other symptoms of ASD include repetitive motor behaviors (such as rocking back and forth), preoccupation with specific subjects, strict adherence to certain rituals, and unusual language use. Up to 30 percent of patients with ASD develop epilepsy, and patients with some forms of the disorder (like Fragile X) also have an intellectual disability. Because it is a spectrum disorder, other ASD patients are very functional and have good-to-excellent language skills. Many of these patients do not feel that they suffer from a disorder and instead think that their brains just process information differently.
Except for some well-characterized, clearly genetic forms of autism (like Fragile X and Rett’s Syndrome), the causes of ASD are largely unknown. Variants of several genes correlate with the presence of ASD, but for any given patient, many different mutations in different genes may be required for the disease to develop. At a general level, ASD is thought to be a disease of “incorrect” wiring. Accordingly, the brains of some ASD patients lack the same level of synaptic pruning that occurs in non-affected people. In the 1990s, a research paper linked autism to a common vaccine given to children. This paper was retracted when it was discovered that the author falsified data, and follow-up studies showed no connection between vaccines and autism.
Treatment for autism usually combines behavioral therapies and interventions, along with medications to treat other disorders common to people with autism (depression, anxiety, obsessive-compulsive disorder). Although early interventions can help mitigate the effects of the disease, there is currently no cure for ASD.
Attention Deficit Hyperactivity Disorder (ADHD)
Approximately three to five percent of children and adults are affected by attention-deficit/hyperactivity disorder (ADHD). Like ASD, ADHD is more prevalent in males than females. Symptoms of the disorder include inattention (lack of focus), executive functioning difficulties, impulsivity, and hyperactivity beyond what is characteristic of the normal developmental stage. Some patients do not have the hyperactive component of symptoms and are diagnosed with a subtype of ADHD: attention deficit disorder (ADD). Many people with ADHD also show comorbidity in that they develop secondary disorders in addition to ADHD. Examples include depression or obsessive-compulsive disorder (OCD). Figure 3 provides some statistics concerning comorbidity with ADHD.
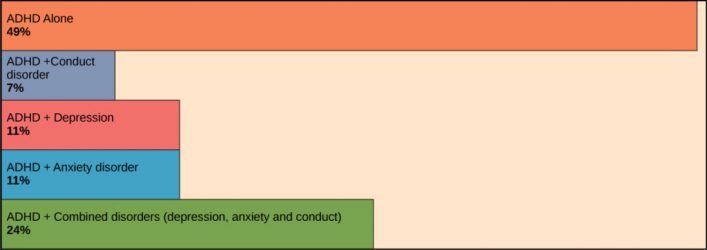
The cause of ADHD is unknown, although research points to a delay and dysfunction in the development of the prefrontal cortex and disturbances in neurotransmission. According to studies of twins, the disorder has a strong genetic component. There are several candidate genes that may contribute to the disorder, but no definitive links have been discovered. Environmental factors, including exposure to certain pesticides, may also contribute to the development of ADHD in some patients. Treatment for ADHD often involves behavioral therapies and the prescription of stimulant medications, which paradoxically cause a calming effect in these patients.
Mental Illnesses
Mental illnesses are nervous system disorders that result in problems with thinking, mood, or relating to other people. These disorders are severe enough to affect a person’s quality of life and often make it difficult for people to perform the routine tasks of daily living. Debilitating mental disorders plague approximately 12.5 million Americans (about 1 in 17 people) at an annual cost of more than $300 billion. There are several types of mental disorders including schizophrenia, major depression, bipolar disorder, anxiety disorders and phobias, post-traumatic stress disorders, and obsessive-compulsive disorder (OCD), among others. The American Psychiatric Association publishes the Diagnostic and Statistical Manual of Mental Disorders (or DSM), which describes the symptoms required for a patient to be diagnosed with a particular mental disorder. Each newly released version of the DSM contains different symptoms and classifications as scientists learn more about these disorders, their causes, and how they relate to each other. A more detailed discussion of two mental illnesses—schizophrenia and major depression—is given below.
Schizophrenia
Schizophrenia is a serious and often debilitating mental illness affecting one percent of people in the United States. Symptoms of the disease include the inability to differentiate between reality and imagination, inappropriate and unregulated emotional responses, difficulty thinking, and problems with social situations. People with schizophrenia can suffer from hallucinations and hear voices; they may also suffer from delusions. Patients also have so-called “negative” symptoms like a flattened emotional state, loss of pleasure, and loss of basic drives. Many schizophrenic patients are diagnosed in their late adolescence or early 20s.
The development of schizophrenia is thought to involve malfunctioning dopaminergic neurons and may also involve problems with glutamate signaling. Treatment for the disease usually requires antipsychotic medications that work by blocking dopamine receptors and decreasing dopamine neurotransmission in the brain. This decrease in dopamine can cause Parkinson’s disease-like symptoms in some patients. While some classes of antipsychotics can be quite effective at treating the disease, they are not a cure, and most patients must remain medicated for the rest of their lives.
Depression
Major depression affects approximately 6.7 percent of adults in the United States each year and is one of the most common mental disorders. To be diagnosed with major depressive disorder, a person must have experienced a severely depressed mood lasting longer than two weeks along with other symptoms including a loss of enjoyment in activities that were previously enjoyed, changes in appetite and sleep schedules, difficulty concentrating, feelings of worthlessness, and suicidal thoughts.
The exact causes of major depression are unknown, and likely include both genetic and environmental risk factors. Some research supports the “classic monoamine hypothesis,” which suggests that a decrease in norepinephrine and serotonin neurotransmission causes depression. One argument against this hypothesis is the fact that some antidepressant medications cause an increase in norepinephrine and serotonin release within a few hours of beginning treatment—but clinical results of these medications are not seen until weeks later. This has led to alternative hypotheses: for example, dopamine may also be decreased in depressed patients, or it may actually be an increase in norepinephrine and serotonin that causes the disease, and antidepressants force a feedback loop that decreases this release.
Treatments for depression include psychotherapy, electroconvulsive therapy, deep-brain stimulation, and prescription medications. There are several classes of antidepressant medications that work through different mechanisms. For example, monoamine oxidase inhibitors (MAO inhibitors) block the enzyme that degrades many neurotransmitters (including dopamine, serotonin, and norepinephrine), resulting in increased neurotransmitters in the synaptic cleft. Selective serotonin reuptake inhibitors (SSRIs) block the reuptake of serotonin into the presynaptic neuron. This blockage results in an increase in serotonin in the synaptic cleft. Other types of drugs such as norepinephrine-dopamine reuptake inhibitors and norepinephrine-serotonin reuptake inhibitors are also used to treat depression.
Other Neurological Disorders
There are several other neurological disorders that cannot be easily placed in the above categories. These include chronic pain conditions, cancers of the nervous system, epilepsy disorders, and stroke. Epilepsy and stroke are discussed below.
Epilepsy
Estimates suggest that up to three percent of people in the United States will be diagnosed with epilepsy in their lifetime. While there are several different types of epilepsy, all are characterized by recurrent seizures. Epilepsy itself can be a symptom of a brain injury, disease, or other illness. For example, people who have an intellectual disability or ASD can experience seizures, presumably because the developmental wiring malfunctions that caused their disorders also put them at risk for epilepsy. For many patients, however, the cause of their epilepsy is never identified and is likely to be a combination of genetic and environmental factors. Often, seizures can be controlled with anticonvulsant medications. However, for very severe cases, patients may undergo brain surgery to remove the brain area where seizures originate.
Stroke
A stroke results when blood fails to reach a portion of the brain for a long enough time to cause damage. Without the oxygen supplied by blood flow, neurons in this brain region die. This neuronal death can cause many different symptoms—depending on the brain area affected— including headache, muscle weakness or paralysis, speech disturbances, sensory problems, memory loss, and confusion. Stroke is often caused by blood clots and can also be caused by the bursting of a weak blood vessel. Strokes are extremely common and are the third most common cause of death in the United States. On average one person experiences a stroke every 40 seconds in the United States. Approximately 75 percent of strokes occur in people older than 65. Risk factors for stroke include high blood pressure, diabetes, high cholesterol, and a family history of stroke. Smoking doubles the risk of stroke. Because a stroke is a medical emergency, patients with symptoms of a stroke should immediately go to the emergency room, where they can receive drugs that will dissolve any clot that may have formed. These drugs will not work if the stroke was caused by a burst blood vessel or if the stroke occurred more than three hours before arriving at the hospital. Treatment following a stroke can include blood pressure medication (to prevent future strokes) and (sometimes intense) physical therapy.
Chapter Summary
The nervous system is made up of neurons and glia. Neurons are specialized cells that are capable of sending electrical as well as chemical signals. Most neurons contain dendrites, which receive these signals, and axons that send signals to other neurons or tissues. Glia are non-neuronal cells in the nervous system that support neuronal development and signaling. There are several types of glia that serve different functions.
Neurons have a resting potential across their membranes and when they are stimulated by a strong enough signal from another neuron, an action potential may carry an electrochemical signal along the neuron to a synapse with another neuron. Neurotransmitters carry signals across synapses to initiate a response in another neuron.
The vertebrate central nervous system contains the brain and the spinal cord, which are covered and protected by three meninges. The brain contains structurally and functionally defined regions. In mammals, these include the cortex (which can be broken down into four primary functional lobes: frontal, temporal, occipital, and parietal), basal ganglia, thalamus, hypothalamus, limbic system, cerebellum, and brainstem—although structures in some of these designations overlap. While functions may be primarily localized to one structure in the brain, most complex functions, like language and sleep, involve neurons in multiple brain regions. The spinal cord is the information superhighway that connects the brain with the rest of the body through its connections with peripheral nerves. It transmits sensory and motor input and also controls motor reflexes.
The peripheral nervous system contains both the autonomic and sensory-somatic nervous systems. The autonomic nervous system provides unconscious control over visceral functions and has two divisions: the sympathetic and parasympathetic nervous systems. The sympathetic nervous system is activated in stressful situations to prepare the animal for a “fight-or-flight” response. The parasympathetic nervous system is active during restful periods. The sensory-somatic nervous system is made of cranial and spinal nerves that transmit sensory information from skin and muscle to the CNS and motor commands from the CNS to the muscles.
*
“Learn By Doing” and “Did I Get This?” Feedback
Learn By Doing 9.1
Which of the following is not a function of the nervous system?
- contribute to homeostatic feedback loops
- stimulate muscles and glands
- produce quick effects by electrochemical mechanisms
- release chemicals into the bloodstream for distribution throughout the body
Learn By Doing 9.2
Below are questions about the relationship between parts of the neuron and their functions. To answer, choose from axon, axon hillock, cell body (soma), axon terminal, or dendrite.
- Which part of the neuron has plasma membrane proteins that will initiate an action potential a long distance away toward its target if the signal reaches a strong enough level called the threshold? axon hillock
- Which part of the neuron has plasma membrane proteins that convert stimuli to graded electrical potentials? dendrites
- Which part of the neuron converts electrical potential to chemical stimuli for other neurons or target effectors? axon terminal
- Which part of the neuron has most of the typical cellular organelles and the nucleus? soma
Learn By Doing 9.3
The function of the whole nervous system is to receive input about changes in the internal and external environment, integrate and coordinate that input, and generate an output. This mirrors the components of homeostatic mechanisms. The single neuron (cell) is well suited because its different parts, dendrites, soma, and axon, serve these functions on a smaller scale.
- Which part of the neuron receives chemical input, either serving as or associated with a “receptor”?dendrites
- Which part of the neuron sums electrical impulses? soma & axon hillock
- Which part of the neuron sends output to the “effector”? axon
Learn By Doing 9.4
What type of glial cell is the resident macrophage within the brain?
- microglia
- astrocyte
- Schwann cell
- satellite cell
What type of glial cell provides myelin for the axons in a tract?
- oligodendrocyte
- astrocyte
- Schwann cell
- satellite cell
What types of macromolecule is the main component of myelin?
- carbohydrates
- proteins
- lipids
- nucleic acids
The main difference between oligodendrocytes and Schwann cells is…
- the type of myelin they produce
- whether one or multiple cells are required to myelinate any axon
- their location
- whether or not they insulate and facilitate signal propagation
Multiple sclerosis is a demyelinating disease affecting the central nervous system. What type of cell would be the most likely target of this disease? Why?
The disease would target oligodendrocytes. In the CNS, oligodendrocytes provide the myelin for axons.
Learn By Doing 9.5
The simulation below allows you to stimulate a neuron and monitor what happens. You can pause, rewind, and move forward in time in order to observe the ions as they move across the neuron membrane. As you explore the stimulation, consider these questions:
What prevents ions from moving across neuron membranes?
What allows ions to move across neuron membranes?
What are gated channels? What are their functions?
How does membrane permeability change because of voltage-gated channels?
What is the sequence of events that generates an action potential?
Learn By Doing 9.6
What ion enters a neuron causing depolarization of the cell membrane?
- sodium
- chloride
- potassium
- phosphate
Voltage-gated Na+ channels open upon reaching what state?
- resting potential
- threshold
- repolarization
- overshoot
What does it mean for an action potential to be an “all or none” event?
Our Answer: The cell membrane must reach threshold before voltage-gated Na+ channels open. If threshold is not reached, those channels do not open, and the depolarizing phase of the action potential does not occur, the cell membrane will just go back to its resting state.
Learn By Doing 9.7
Which of the following is probably going to propagate an action potential fastest?
- a thin, unmyelinated axon
- a thin, myelinated axon
- a thick, unmyelinated axon
- a thick, myelinated axon
Learn By Doing 9.8
The binding of a neurotransmitter to the post-synaptic membrane opens which type of channel?
- mechanically-gated
- ligand-gated
- voltage-gated
What happens to a postsynaptic cell membrane if chloride channels open as a result of neurotransmitter binding?
- The cell membrane depolarizes.
- IPSP (inhibitory postsynaptic potential) is achieved.
- EPSP (excitatory postsynaptic potential) occurs.
- The cell membrane repolarizes.
Hint: Negatively charged chloride ions are more concentrated outside the cell membrane and will follow their concentration gradient to diffuse across an open membrane channel.
Is the entry of calcium into the presynaptic axon terminus an active or passive process? Explain.
It is a passive process (diffusion). Calcium concentration is higher in the interstitial spaces around the neuron.
Would a spinal nerve have afferent or efferent transmission or both? Explain your reasoning.
The posterior root has afferent (sensory) transmission, and the anterior root has efferent (motor) transmission. Since spinal nerves are formed by the fusion of these posterior and anterior spinal rootlets, they would have both transmissions within that mixed nerve.
Learn By Doing 9.9
Which of the following is true only about action potentials but not about graded potentials?
- inhibitory or excitatory
- can decrease in strength over time
- graded
- uses voltage-gated channels
- reversible
Hint: Graded potentials are also known as local potentials that can initiate the propagation of an action potential that travels one direction from the trigger zone to the axon terminal very quickly.
Why should the opening of a K+ channel cause hyperpolarization?
Due to the concentration gradient of the ion, K+ diffuses out of the cell and hyperpolarizes the cell membrane.
Both graded potentials and action potentials can involve Na+ and K+ channels. What is different in how these channels behave that creates the different types of potentials?
Our Answer: Graded potentials will open or close Na+ and K+ channels in response to various stimuli but when they do they create only small localized changes in membrane potential. For an action potential, a voltage-gated Na+ channel opens in response to voltage change and causes a standardized large and quick change in membrane potential, and K+ channels open slower while Na+ channels close. This sets up the propagation of action potentials sequentially along the axon at the same strength in one direction.
The channels responsible for EPSPs are sodium channels, while those responsible for IPSPs are potassium and chloride channels. Can you explain why?
Our Answer: Due to the higher extracellular concentration of Na+, opening Na+ channels causes rapid inward diffusion of Na+ that leads to depolarization (less negative or positive on the inside) of the cell membrane. On the other hand, the opening of K+ channel or the Cl–-channel causes these ions to follow the concentration gradient out of the cell (K+) or into the cell (Cl–), respectively, leading to hyperpolarization (even more negative than resting potential on the inside).
Learn By Doing 9.10
In addition to meninges, fluid-filled spaces help protect the brain’s nervous tissue. The subarachnoid space would be found between which two meninges?
- dura mater and arachnoid mater
- arachnoid and pia mater
- dura mater and pia mater
Learn By Doing 9.11
Which part of the brain has noticeable gyri and sulci that increase surface area for cortical gray matter?
- pons
- brainstem
- diencephalon
- left and right hemispheres
Which statement is true of grey matter?
- It is the primary source of intelligence
- It is located mainly in the frontal lobes
- It processes information
- It is the dominant type of nervous tissue in the brain
Which structure predominates in the white matter of the brain?
- myelinated axons
- neuronal cell bodies
- ganglia of the parasympathetic nerves
- bundles of dendrites from the enteric nervous system
Which area of the brain is not part of the cerebral cortex?
- Frontal lobe
- Cerebellum
- Parietal lobe
- Temporal lobe
Which part of the cerebral cortex contains the visual center?
- temporal lobe
- frontal lobe
- parietal lobe
- occipital lobe
Learn By Doing 9.12
Which part of the brain includes the hippocampus and amygdala and is involved in emotions and memory?
- precentral cerebral gyrus
- reticular activating formation
- postcentral cerebral gyrus
- limbic system
Learn By Doing 9.13
Afferent means “toward,” as in sensory information traveling from the periphery into the CNS. Efferent means “away from,” as in motor commands that travel from the brain down the spinal cord and out into the periphery. Would a spinal nerve have afferent or efferent transmission or both? Explain your reasoning.
Our Answer: The posterior root has afferent (sensory) transmission, and the anterior root has efferent (motor) transmission. Since spinal nerves are formed by the fusion of these posterior and anterior spinal rootlets, they would have both transmissions within that mixed nerve.
Learn By Doing 9.14
Each of the following neurons (cells) are part of the nervous system. Based on the description of its location and function, classify each as belonging to either the Central Nervous System division (CNS) or the Peripheral Nervous System division (PNS):
- Neuron is associated with thermoreceptor in the skin. PNS
- Neuron is associated with nasal chemoreceptors. PNS
- Neuron is associated with internal thermoreceptors near the hypothalamus. CNS
- Neuron is located in the outer cortex of the brain, getting sensory input and initiating the voluntary override that prevented you from dropping the pan. CNS
- Neuron is located in the hypothalamus, receiving input and initiating response to hypothermia or hyperthermia. CNS
- Neuron is located in the spinal cord, transferring sensory input to motor output for reflex, such as the withdrawal reflex when a finger touched a hot pan. CNS
- Neuron is carrying motor stimulus to arm muscle to pull away from hot pan. CNS
- Neuron is carrying motor stimulus to arm muscle, overriding reflex to hold onto the pan a little longer. CNS
- Neuron is carrying motor stimulus to sweat gland in skin to stimulate sweating and cool off body. CNS
- Neuron is carrying motor stimulus to skeletal muscles to stimulate shivering to warm body. CNS
Which of the following isnotassociated with the Peripheral Nervous System?
- motor output to skeletal muscles
- motor output to glands, smooth muscle, and cardiac muscle
- integration of sensory input, and initiation of voluntary or involuntary output
- sensory input from peripheral receptors
The fight-or-flight response is controlled by the…
- autonomic nervous system
- somatic nervous system
- fast nervous system
- sympathetic nervous system
For each of the statements below, decide which branch of the autonomic nervous system is likely to be involved.
- Faster blood flow and more blood flow to skeletal muscles
sympathetic - Stimulation of sweat glands to reduce excessive body heat
sympathetic - A good internal body state sitting down to a hearty meal
parasympathetic